Traumatic Brain Injury in Children: Critical Care Management
56.1 Epidemiology
Traumatic brain injury (TBI) remains the leading cause of death between the ages of 1 and 18 years. In the United States, one TBI has been reported to occur as frequently as every 15 seconds, with one death resulting every 12 minutes. According to the Centers for Disease Control, each year in the United States approximately 1 million infants, children, and young adults sustain a TBI. Of these, 600,000 will come to medical attention and 250,000 will be admitted to the hospital; 30,000 will be permanently disabled and 7,000 will die. Severe TBI in children is responsible for nearly $20 billion annually in health care costs. International efforts to define the mortality and morbidity burden of severe TBI have proved fruitful. Aggressive prevention efforts and new insights into the pathophysiology of primary injury (moment of impact), as well as the evolution and reduction of secondary injury, continue to show great promise. However, only in the past 15 years has direct scientific inquiry begun to address the question of the optimal treatment of severe TBI in children.
56.2 Development of International Standards: Evidence-Based Management Guidelines and Common Data Elements
In 1996, Bullock and colleagues,1 using the principles of contemporary evidence-based medicine, published the first guidelines for the management of adult severe TBI. Before this seminal effort, which included the input of international experts, the management of severe TBI in adults was found to be highly heterogeneous, colloquial, and not evidence-based. The panel reviewed studies relevant to the management of head-injured patients and stratified the data based on the quality of evidence. The stratification of existing head injury–related publications according to quality yielded a summary of practical, weighted consensus treatment guidelines and assisted in the charting of directions for future research and funding efforts. Although international acceptance and application were initially variable, independent evaluations of the impact of the application of these guidelines have consistently demonstrated significant reductions in mortality, adjusted length of stay, and costs.2,3
In 2000 and again in 2012, similarly configured panels of pediatric experts were convened to develop consensus guidelines for the treatment of severe TBI in infants, children, and young adults.4–23 The developing brain has unique anatomical and cerebrovascular characteristics that warranted the development of independent guidelines. Additionally, the response to trauma of pediatric patients—particularly infants and young children—mandates that they not be treated as “little adults.” The evidence-based approach was reapplied to the relevant literature on pediatric brain trauma, and “Guidelines for the Acute Medical Management of Severe Traumatic Brain Injury in Infants, Children, and Adolescents” was published. Three degrees of evidence quality were applied throughout the process (see box “▶ Evidenced-Based Medicine Levels of Evidence in Severe Traumatic Brain Injury”), and three classes of evidence were identified (see box “▶ Classification of Evidence in Severe Traumatic Brain Injury”). In the most recent edition, the pediatric guidelines encompass 15 topics deemed to be imperative to improving outcome. These topics form the scaffolding for this chapter.
Evidenced-Based Medicine Levels of Evidence in Severe Traumatic Brain Injury
Level 1
Sufficient evidence exists that these therapies “should be done.”
Level 2
Sufficient evidence exists that these therapies “should be considered.”
Level 3
Sufficient evidence exists that these therapies “may be considered.”
Classification of Evidence in Severe Traumatic Brain Injury
Randomized controlled trials completed; may still lack sufficient numbers and have methodologic inadequacies
Clinical studies based on clearly reliable data; prospective collection with retrospective analysis acceptable
Retrospective analyses; case series/reports, databases, registries, expert opinion
During the past several decades, it has been recognized that the analysis of clinical studies in TBI has been hampered by a lack of clarity in the definitions of patient characteristics and by inconsistencies in reporting important factors between studies. Specifically, despite the best intentions of authors to fully describe (1) the population under study, (2) the clinical variables collected, and (3) the outcomes that were observed, comparisons of the results of clinical studies suffered from ad hoc editorial decisions made during manuscript preparation. In seminal work on this topic, Lingsma and colleagues took several years to painstakingly combine data from 10 randomized controlled trials (RCTs) and three observational trials in the International Mission on Prognosis and Clinical Trial in Traumatic Brain Injury (IMPACT) study.24 This yielded an analysis of 9,578 patients enrolled at 265 clinical sites that found striking differences in unfavorable outcome rates between centers in the United States and Europe. This led Maas and colleagues to develop common definitions of various aspects of TBI care, the so-called common data elements (CDEs), to improve the uniformity of defining TBI care and data collection.25 In pediatric TBI, CDEs for acute data collection, imaging, biomarkers, and outcomes were recently published in liaison with the National Institute of Neurological Disorders and Stroke (NINDS), and these elements will be required for future funded clinical trials.26–29
The goals of contemporary pediatric neurocritical care are the early identification and management of surgically evacuable lesions and the prevention of secondary injury. Pediatric neurocritical care strategies for severe TBI have evolved from strategies for exclusively supportive care to those attempting to (1) optimally match substrate delivery and cerebral metabolism with the use of multimodal monitoring, (2) prevent herniation by anticipatory clinical strategies and meticulous nursing care, and (3) target specific mechanisms involved in the evolution of secondary injury with novel or experimental therapy. The re-evaluation of current approaches, the role of newer technologies, and the differences between adults and children will be highlighted.
56.3 Clinical Presentation
The rapid identification of injuries and the assessment of their severity are essential to minimize the risk for undertreating evolving clinical conditions and to ultimately optimize outcomes. The Glasgow Coma Scale (GCS) score30 (▶ Table 56.1), first described in 1974, remains a critical tool for communicating the severity of neurologic injury after TBI. Hypoxemia, shock, and hypotension all reduce the GCS score. Optimal resuscitation, therefore, is an important prerequisite to assigning the best GCS score. In preverbal children, the GCS score has been modified to allow a score of 5 for the cooing child. The modified GCS score for infants and children (▶ Table 56.1), although convenient, has not been subjected to extensive peer review.31,32 For this reason, the infant GCS score is best applied by those familiar with the management of young children. The best motor score is the most predictive component of the GCS.33 Additionally, the AVPU (awake, [responds to] verbal, [responds to] pain, unresponsive) scale provides a rapid, albeit crude, assessment of neurologic status. Moreover, the pupillary examination is an essential aspect of the physical examination to triage children with TBI.34 In a rigorous study of 500 normal subjects, Meyer et al demonstrated that anisocoria of less than 1 mm is clinically unimportant. Sparse evidence exists regarding the prognostic significance of the light reflex. Of note, hypotension, hypothermia, and hypoxemia all confound the pupillary light reflex.35 Optimal resuscitation, therefore, remains the clinical linchpin on which all other assessments, diagnoses, and prognoses rest.
Original | Modified for infants | |
Best eye response |
|
|
Best verbal response |
|
|
Best motor response |
|
|
The accurate assessment of the child with TBI is essential to developing a comprehensive care plan that includes the necessary imaging, invasive procedures, and medical and surgical therapies. To date, this has largely been based on the physical findings and the GCS score, as outlined above. In particular, the GCS score combined with signs of acute brain dysfunction aid in the assessment of patient acuity. Clinical signs such as persistently impaired consciousness, a GCS score falling by 2 or more points, amnesia, focal deficits, and evidence of skull fracture all necessitate rapid triage.36–39 However, an increasing body of literature is starting to question the assumption that the severity of TBI must be tied to these classic signs40 and have suggested that imaging and other factors may add specificity to predicting the need for interventions and outcomes.
56.4 Initial Resuscitation and Prehospital Stabilization
Once a child has sustained severe TBI, rapid assessment, stabilization with triage, and transfer to the highest-necessary level of care are the next steps. The centralization of pediatric trauma–specific resources is the natural extension of this axiom, which, along with patterns of resource allocation, are currently in search of validation. Olson and colleagues41 published one of the first studies to suggest that the centralization of resources, coupled with an urgency to transfer patients as quickly as possible, might be an incompletely developed model. Their historically controlled review found an increase in trauma-related deaths after the implementation of the Oregon State Trauma System. Patients injured in a rural setting were transferred to a higher level of care, incurring longer transport times. It appears that a system intended to facilitate rapid transfer may have resulted in premature transfer.
Studies of transport decisions after severe TBI in children have been largely retrospective, reporting data on practice patterns. A prospective review of transport decisions comparing children who had severe TBI and were directly referred to an urban Level 1 pediatric trauma center with those who arrived by indirect referral42 demonstrated that those who had severe TBI (GCS score ≤ 8) and were directly referred had better survival. These limited data, along with information from adult TBI studies, allowed the first edition of the pediatric TBI guidelines19 to recommend the transfer of children with severe injuries to centers that had sufficient experience with such patients.
Regardless of transportation from the scene of the injury, several studies have consistently demonstrated increased morbidity and mortality due to failure to correct hypotension and hypoxemia or evacuate mass lesions.43–45 Gentleman reported during an 11-year period in England that an approximately eightfold increase in endotracheal intubation yielded a 64% reduction in hypoxemic episodes.46 This was accompanied by a 40% reduction in the mortality rate and a 45% increase in good outcome. Although the etiology of this improvement may be multifactorial, early, targeted intervention must be the objective of initial therapy.
The success of this study and others further reinforced the importance of reliable, early airway protection.47 A provocative study by Gausche and colleagues,48 however, added further clarity to the issue. In an RCT, airway management with bag valve mask (BVM) ventilation was compared with airway management with tracheal intubation in the prehospital setting in two urban pediatric trauma centers. There was no demonstrable advantage to tracheal intubation in the only prospective study to date. The severe TBI subgroup analysis (8 of 25 treated with BVM vs. 9 of 36 treated with tracheal intubation) showed that a “good” neurologic outcome was independent of how airway control was accomplished. The subgroup analysis suggests that aggressive airway management after severe TBI may not be essential. Further studies are needed to validate this potentially underpowered result and to address the question over a range of transport times.
The prehospital tracheal intubation of infants and children requires specialized training. There is currently little evidence that aggressive prehospital airway management changes the outcome for children or adults after severe TBI,4 and this topic is not explicitly addressed in the newest version of the guidelines.49 All trauma patients with supraclavicular injury should be assumed to have cranial and cervical spine injuries until proven otherwise. The initial evaluation of a child after severe TBI begins with a demonstration by an experienced clinician that the child has a patent, maintainable airway. This, by definition, requires that the patient be conscious, alert, and breathing spontaneously. All unconscious patients are assumed to have an obstructed airway requiring immediate airway evaluation. (It will be helpful for readers if we define “unconscious” as not following commands, a Glasgow Coma Score of 8 or less, and not opening the eyes or speaking.)
The relatively larger head, occiput, and tongue of an infant, coupled with the shorter, narrower epiglottis, facilitate airway obstruction if the child’s sensorium has been clouded by a concussive injury. The rescuer must alleviate obstruction immediately (while protecting the cervical spine) to minimize secondary injury due to hypoxemia.
The mnemonic SOAP has been used for the necessary components of an optimal preparation to secure the airway.
S (suction). For most patients, a flexible 10F catheter will suffice. However, for school-aged children (older than 5 years), we recommend a rigid, plastic suction catheter that can remove particulate debris. This nonkinking catheter allows direct oropharyngeal suctioning. The suction device should be able to provide 30 L/min or greater and a vacuum of 300 mm Hg when clamped.
O (oxygen). Oxygen (FiO2 = 1.0) should be delivered immediately to the patient before intubation. Optimal positioning of the patient requires that axial in-line immobilization be maintained to stabilize the cervical spine. The delivery of 100% oxygen facilitates the washout of nitrogen from the functional residual capacity, allowing adequate alveolar oxygenation for safe intubation of the trachea.
A (airway). Once the patient is properly positioned, ventilation and oxygenation are controlled, and an age-appropriate laryngoscope blade and endotracheal tube have been selected, the patient is ready to be intubated. Secure the endotracheal tube with adhesive tape, but refrain from passing the tape circumferentially around the neck because compression of the cerebral venous return may occur.
P (pharmacology). The medications chosen must be potent and rapid in their onset of action. The goals of analgesia, amnesia, and neuromuscular blockade must be met rapidly. Ideally, the patient never receives a preintubation positive-pressure breath.
Optimal tracheal intubation of the child with severe TBI requires a cerebroprotective, rapid-sequence induction technique whenever possible. BVM positive-pressure ventilation is to be avoided unless hypoxemia or impending herniation is suspected. One study50 suggested that the BVM technique may cause more unintentional cervical spine manipulation than previously appreciated, so great care is advised during manual ventilation.
If a victim of head trauma meets any of the following criteria, assisted ventilation is indicated, with an orotracheal tube as the modality of choice:
GCS score of 8 or lower
Decrease in the GCS score of more than 3, independently of the initial GCS score
Anisocoria of more than 1 mm
Cervical spine injury compromising ventilation
Apnea
Hypercarbia (PaCO2 > 45 mm Hg)
Loss of pharyngeal reflex
In children, the recommended route of initial airway control is orotracheal intubation under direct vision.51 Nasotracheal intubation should be avoided in children with severe TBI for several reasons, including that blind passage of the endotracheal tube around the acute pediatric nasopharyngeal angle makes this procedure an unnecessary obstacle to rapid, physiologic resuscitation and risks injuring the underlying brain in cases of occult skull base fractures or sinus injuries. Orotracheal intubation can be accomplished with a two-person strategy that protects the cervical spine from injury. Although a normal lateral cervical spine roentgenogram is reassuring, it does not rule out cervical spinal injury.52 Spinal immobilization must be maintained. One operator accomplishes this via in-line cervical immobilization while the second clinician intubates the trachea. Care must be taken during positioning in preparation for intubation in infants to avoid pressing into the soft tissues of the submental region and accompanying strap muscles because inadvertent airway obstruction will ensue.
56.4.1 Rapid-Sequence Induction
Tracheal intubation, although a life-saving procedure, remains a potent, noxious stimulus that can alter cerebral hemodynamics. Rapid-sequence induction safely secures the airway of an unprepared patient at risk for aspiration of gastric contents. There is minimal resistance to direct laryngoscopy, and the normal responses to having a large foreign body intentionally placed into the trachea are eliminated. Rapid-sequence induction has been repeatedly documented as a safer technique than either nasotracheal intubation or orotracheal intubation without neuromuscular blockade.53,54
In the head-injured pediatric patient, a cerebroprotective rapid-sequence induction strategy should be employed. The sequence involves preparation, preoxygenation, sedation, neuromuscular blockade, and orotracheal intubation. Pharmacologic adjuncts are used to avoid the morbidity associated with hypotension, hypoxemia, intracranial hypertension, and gastric aspiration. The neurologic and hemodynamic status of the patient will direct the pharmacologic strategy. For the victim in cardiac arrest, cardiopulmonary resuscitation should begin immediately. No pharmacologic adjuncts are necessary to secure the airway. For the hemodynamically unstable patient, the combination of lidocaine, fentanyl, and vecuronium (▶ Table 56.2) is a safe and effective strategy. For the hemodynamically stable patient, the same sequence of drugs in addition to a rapidly acting benzodiazepine (midazolam) can be successfully used. Etomidate has become an effective agent for intubating the head-injured child. In hemodynamically stable patients, doses of 0.2 to 0.3 mg/kg reduce intracranial pressure (ICP) predictably while preserving the mean arterial pressure. Some reports have suggested an increased risk for adrenal insufficiency with etomidate in children with shock.55,56
Situation | Drugs |
Cardiopulmonary arrest | Resuscitation drugs |
Hemodynamically unstable | Etomidate, 0.1–0.2 mg/kg Fentanyl, 2–5 µg/kg |
Lidocaine, 1 mg/kg | |
Vecuronium, 0.3 mg/kg | |
Hemodynamically stable | Etomidate, 0.3 mg/kg Fentanyl, 2–4 µg/kg |
Lidocaine, 1 mg/kg | |
Diazepam or midazolam, 0.1–0.2 mg/kg | |
Vecuronium, 0.3 mg/kg | |
Evidence of intracranial hypertension (and hemodynamically stable) | Etomidate, 0.1–0.2 mg/kg Fentanyl, 2–4 µg/kg Lidocaine, 1 mg/kg Thiopental, 4–5 mg/kg Vecuronium, 0.3 mg/kg |
An alternative in the hemodynamically stable head-injured patient is thiopental, an ultrafast-acting thiobarbiturate. Thiopental reduces the cerebral metabolic rate for oxygen by 45 to 50% within 15 seconds of intravenous injection. This in turn attenuates the intracranial hypertension associated with direct laryngoscopy. The high lipophilicity of thiopental results in rapid cerebral wash-in and equally rapid washout. Therefore, although thiopental is an excellent agent for facilitating the rapid-sequence induction of anesthesia, it must be followed with another sedative–analgesic agent, although its availability in the United States has been limited in recent years. Fentanyl, a short-acting narcotic, and lidocaine reduce the catecholamine surge associated with direct laryngoscopy.
56.4.2 Circulatory Stabilization
The assessment of circulatory function after trauma involves a rapid determination of heart rate, blood pressure, central and peripheral pulse quality, skin perfusion, and cerebral perfusion. The identification and correction of airway obstruction, inadequate ventilation, and shock take priority over a detailed neurologic assessment. The first priority in managing the head-injured patient is complete, rapid physiologic resuscitation. Hypoxemia and hypotension must be avoided during resuscitation.43 Children who sustain age-specific hypotension (▶ Table 56.3) have a worse outcome in comparison with their normotensive peers.57,58 Mild systemic hypertension early after TBI may be beneficial and has been associated with favorable outcomes. Although intracranial hypertension and cerebral herniation are the major complications of severe TBI, brain-specific interventions in the absence of signs of herniation or other neurologic deterioration are not currently recommended. Moreover, interventions designed to manage malignant intracranial hypertension (e.g., osmotherapy or diuretics) may be counterproductive to initial resuscitative efforts.
Age range (y) | Hypotension (mm Hg) |
0–1 | < 65 |
2–5 | < 75 |
6–12 | < 80 |
13–16 | < 90 |
Posttraumatic hypotension must be assumed to be hypovolemic (i.e., hemorrhagic) in nature, but it may also have a component of myocardial depression due to blunt cardiac injury. Blunt cardiac injury, however, is not as common in children as it is in adults.59 Fluid therapy in hypovolemic shock is based on the principle of replacement of large volumes as rapidly as tolerated of whatever the patient is losing. The choice of which fluid to use has been a source of controversy. The current recommendation is 20 mL of isotonic crystalloid per kilogram given intravenously. Hypotonic fluid is to be avoided in the initial empiric resuscitation of the brain-injured patient. Subsequent doses of fluid should be more targeted based on the nature of fluid loss (i.e., packed red blood cells) and should be isotonic.
In summary, there is no Level 1 evidence to fully define optimal prehospital strategies. There is substantial evidence (generally categorized as Level 2 within the guidelines) to suggest that the administration of supplemental oxygen, avoidance of hypoxia and hypotension, early airway protection, and rapid fluid resuscitation are associated with improvements in outcome. Establishing a secure airway with sedative–analgesic agents and neuromuscular blockade and without causing secondary insults is essential in children who have severe injuries. The development of local emergency medical service and hospital evidenced-based protocols for achieving these goals is recommended, along with efforts to improve quality.
56.5 Diagnostic Studies
56.5.1 Computed Tomography
From its first moment of commercial availability in 1973, computed tomography (CT) has had an enormous beneficial impact on neurocritical care. A three-dimensional anatomical map of the brain structure facilitates diagnosis and management decisions in children with severe TBI. This modality, however, is not without limitations and must be utilized as one, albeit important, piece of information. After severe TBI, approximately 15% of adults with a normal CT scan will develop significant intracranial hypertension. Similarly, patients with normal initial CT scans but who have hypotension or abnormal posturing have the same propensity to develop intracranial hypertension as their counterparts with abnormal scans.60,61
For children, there have been several recent advances in the field of imaging. Figg and colleagues found that repeated CT in children who did not have neurologic deterioration was not needed, leading to a new Level 3 recommendation within the guidelines.62 More provocatively, after studying a population of more than 40,000 children, Kuppermann and colleagues developed decision rules regarding when imaging is needed based on history and physical examination for children in the emergency department.63 Importantly, this study was intended to limit the exposure of uninjured children to ionizing radiation, and it should not be extrapolated to children at high risk for severe injuries.
56.5.2 Monitoring of Cerebral Blood Flow
Techniques for the determination of cerebral blood flow monitoring after severe TBI include (1) stable xenon–enhanced CT, (2) radioactive (inhaled or injected) 133Xe methods, and (3) transcranial Doppler methods.
Stable xenon CT cerebral blood flow measurement can aid in clinical decision making in the management of infants and children with severe TBI. This valuable technique was in clinical use but was temporarily removed from clinical use by the U.S. Food and Drug Administration (FDA). It is once again available as a research tool with investigational new drug (IND) approval by the FDA, but its use is very sporadic at this time. Although not a “monitor” in the sense that it does not provide a minute-to-minute assessment of changes, stable xenon CT blood flow measurement provides important information about regional cerebral blood flow and its relationship to anatomical disturbances. This information can be readily coupled to nearly all CT scans obtained in the evaluation and follow-up of severely brain-injured infants and children, including the initial scan. The procedure can be completed in a relatively short time (usually within 30 minutes) and is technically contraindicated only if the FiO2 or mean airway pressure is high (because of the need for the inhalation of 50% xenon gas, which has the effect of inherently increased density) or if the ICP is markedly increased. Serial stable xenon CT cerebral blood flow measurements can be coupled to a physiologic manipulation, such as altering the mean arterial blood pressure or PaCO2. These dynamic “before and after” studies often provide additional insight into the optimal titration of bedside interventions and further prognostic information. In the largest series to date, we found that unfavorable outcome after severe TBI was associated with early decreases in cerebral blood flow to 20 mL/100 mg per minute or less and loss of CO2 autoregulation measured with stable xenon CT imaging.64 Although a promising modality, it has yet to make an impact on the routine care of children with severe TBI.
Obrist and colleagues65 pioneered application of the 133Xe method to assessment of patients after TBI. With multiple detectors, this method can provide information on regional cerebral blood flow and can be used in dynamic studies. Its advantages over the stable xenon CT method are that it can be frequently repeated, and it is a bedside technique. This method has been used in children with TBI to provide important descriptive information. However, its inability to correlate flow with anatomical disturbances is an important limitation.
Transcranial Doppler, a technique that uses sound waves to measure the blood flow velocity in intracerebral vessels, is frequently used to screen for vasospasm after spontaneous subarachnoid hemorrhage. In pediatric TBI, this modality has been used in several research applications to determine autoregulation, CO2 reactivity, and hyperemia.66–68 Given its noninvasive nature, future research may expand the routine role of transcranial Doppler in pediatric TBI.
56.5.3 Monitoring of Cerebral Metabolism
The jugular venous saturation has been used extensively to monitor cerebral oxygen delivery in adults, and there has been recent interest in assessing its utility in children.69 Studies in adults suggest that therapies like barbiturates and hyperventilation can be effectively titrated to jugular venous saturation. Gopinath et al reported the association of desaturation below the threshold value of 50% and mortality in adults.70 Jugular venous desaturation below this level was rarely the sole indication that urgent intervention was necessary. Technical problems with placement and false desaturation readings are two additional caveats to this technique. Nevertheless, this monitoring tool can provide valuable information to assist in clinical decision making.
Furthermore, transcranial oximetry has been described as a noninvasive technique that may prove quite useful. Dunham and colleagues71 reported that measurement of the transcranial oxygen saturation (StcO2) detected cerebral hypoxemia even in the context of a cerebral perfusion pressure (CPP) of 70 mm Hg or higher in 16% of their nearly 4,000 observations over 6 days in a neurosurgical intensive care unit. Finally, brain tissue oxygen pressure (PbO2) correlated with cerebral hypoperfusion, GCS score, severity of injury, and mortality. Further studies are needed to confidently and practically import these techniques to the bedside.
56.5.4 Monitoring of Intracranial Pressure and Brain Oxygen Pressure
It has long been recognized that clinical signs like pupillary size, light response, and papilledema fail as early indicators of intracranial hypertension. Most (but not all) patients at risk for the development of intracranial hypertension are identified by CT. ICP monitoring devices provide a window into the global pressure of the brain and impending crises—when the ICP reaches levels that can cause cerebral ischemia or herniation. ICP monitoring can be accomplished with either intraparenchymal or intraventricular devices. The preferred device remains the ventriculostomy catheter, which facilitates the real-time monitoring of ICP and affords the clinician the option of therapeutically draining cerebrospinal fluid (CSF). In the ideal circumstance, infection rates are acceptably low. Currently, ICP monitoring by ventricular catheter is considered the most accurate, inexpensive, and reliable method. The ventricular catheter also affords a key therapeutic option—CSF drainage. Other acceptable methods include parenchymal fiberoptic and microtransducer systems, whereas subarachnoid, subdural, and epidural monitors of any type are less reliable. Although the fiberoptic ICP catheter is useful in cases of severe intracranial hypertension with slitlike ventricles, data drift after 4 to 5 days may limit its use beyond the first week after injury.72 Furthermore, currently available fiberoptic catheters are single-calibration devices. Recently, an approach in which both an externalized ventricular drain (for drainage of CSF as a therapeutic maneuver) and an intraparenchymal device (for continuous measurement of ICP) are placed has been advocated.73
Although there is currently insufficient evidence to support an absolute standard regarding when to place an ICP monitoring device, Level 3 evidence32,74–79 supports the placement of a device in patients with a GCS score of 8 or lower. ICP monitoring is considered a reasonable option for infants (even those with open fontanels) and children with severe TBI and a GCS score of 8 or lower. The threshold of when to treat an elevation is currently any sustained ICP of 20 mm Hg or higher, again supported with Level 3 evidence.80 Age-specific recommendations are currently not possible until our understanding of ICP, CPP, and the intricacies of cerebral hemodynamics are clarified in coming years. The range of 45 to 60 mm Hg currently represents the closest age-related goals for CPP.81 The identification of a “herniating pressure” and its relationship to both ICP and CPP remains critical to our understanding of this parameter. Moreover, the insidious nature of cerebral hypoxemia warrants the integration of multimodal measures of cerebral hemodynamics. ICP monitoring was also suggested to be appropriate in adults with severe TBI and a normal head CT scan if hypotension or motor posturing complicated the clinical course. This approach appears reasonable in children as well. The risks versus benefits of ICP monitoring must be considered in the clinical decisions for patients in whom the complication rate is high, such as those with coagulopathy. Based on recent work with recombinant factor VIIa, this agent may rapidly minimize the risk for bleeding in patients with a coagulopathy after severe TBI.82
Because of the high risk for cerebral hypoxia and/or ischemia,83 the measurement of cerebral oxygen after TBI has garnered intense interest for some decades. Most recently, FDA-approved catheters that measure interstitial brain oxygen (partial pressure of brain oxygen, or PbO2) have been introduced into the clinical milieu. Several reports have demonstrated some utility in PbO2 monitoring.84–89 A report by Stiefel and colleagues showed that a protocol targeting a PbO2 of at least 25 mm Hg decreased mortality compared with mortality in historical controls for adult TBI victims. In children, several studies suggest that PbO2 monitoring may be helpful. Figaji and colleagues demonstrated that the maintenance of conventional management targets (ICP < 20 mm Hg, CPP ≥ 50 mm Hg, PaO2 ≥ 60 mm Hg, SaO2 ≥ 90%, and hemoglobin ≥ 8 g/dL) resulted in at least one episode of PbO2 below 20 mm Hg in 80% of children and episodes of PbO2 below 10 mm Hg in 32% of children,90 and that children with these episodes had an increased incidence of unfavorable outcome in multivariate regression analysis. Steifel and colleagues demonstrated that decreased PbO2 was associated with ICP and CPP derangements.91 A decisive study demonstrating that PbO2 monitoring (or a PbO2 threshold, more likely) improves neurologic outcome after pediatric TBI has yet to be performed. However, this technology, with relatively low risk and the potential to effect significant changes in clinical strategies, has been adopted within more centers over the last several years.
56.6 Medical Treatment of Elevated Intracranial Pressure
56.6.1 Hyperosmolar Therapy
Basic science support for the impact of osmotherapy is over a century old,92 with an ever-expanding variety of agents being introduced into the clinical armamentarium. First mannitol and then various hypertonic saline solutions have been considered an integral part of neurocritical care for pediatric TBI for years despite few definitive trials demonstrating efficacy in improving overall outcome. In fact, like many aspects of TBI care, mannitol administration is so ingrained within standards of care protocols that RCTs comparing mannitol with placebo are extremely difficult to design. It may be that other study designs, such as comparisons of established therapies based on comparative effectiveness research strategies, could play some role in establishing superior strategies. Pathophysiologically, the immediate benefit of osmotherapy in the context of raised ICP is undeniable; an immediate reduction in blood viscosity leads to decreases in cerebral blood volume and ICP via Poisseuille’s law. This results in an immediate reduction in ICP, albeit a transient one. Muizelaar and colleagues93 demonstrated that this mechanism (viscosity autoregulation) appears to operate only when pressure autoregulation of the cerebral blood flow is intact. When it is not intact, the decrease in viscosity will be accompanied by an increase in flow and no change in vessel caliber, cerebral blood volume, or ICP. Note that if a bolus of mannitol is given too rapidly and produces transient systemic hypertension in a patient with defective pressure autoregulation, cerebral blood volume and ICP may transiently increase. More prolonged decreases in ICP are observed after the administration of mannitol and are related to the dehydration of brain parenchyma via an osmotic effect. Theoretically, this osmotic effect should operate only where the blood–brain barrier is intact, although there is some controversy regarding the location(s) of the dehydrating effect of mannitol.94 It must be emphasized that osmolar therapy should be carefully titrated with careful attention to the maintenance of a euvolemic state. Experimental evidence indicates that a high serum osmolarity (serum osmolarity > 320 mOsm/L) may be associated with renal failure in adults, thus limiting the use of mannitol under these conditions.
Hypertonic (3%) saline has become a popular alternative to mannitol, and an increasing body of literature supports its use to mitigate ICP crises. The start of this effort can be traced back to Peterson and colleagues,95 who reviewed their experience with 68 children from 1985 to 1990. Their clinical protocol incorporated conventional therapy with intentional osmotherapy combining 3% saline infusion, furosemide, and mannitol. They showed effective ICP control for the majority of the patients, with no hyperosmolarity complications. Two studies using hypertonic saline solutions were judged to be Level 2 evidence in the most recent guidelines. Fisher and colleagues randomized 18 children to receive 3% normal saline solution or 0.9% normal saline solution to determine the effect of the intervention on ICP (> 2 hours after administration), ultimately finding that the experimental group had decreased ICP and a need for additional therapies.96 Similarly, Simma and colleagues tested the hypothesis that 1.7% normal saline solution (administered as a continuous infusion over 3 days) would decrease ICP compared with lactated Ringer solution.97 They failed to show an effect on overall ICP but did demonstrate a decreased need for other ICP therapies in the experimental group. Khanna and colleagues98 reported their experience in 10 children with malignant intracranial hypertension refractory to conventional therapy. Doses ranging from 0.1 to 1.0 mEq of sodium per kilogram per hour were titrated to achieve a targeted serum sodium level. Targeted osmotherapy resulted in lower average ICP, increased CPP, less frequent ICP spikes, and predictable increases in serum sodium and osmolarity. One child required continuous venovenous hemofiltration. Over time, his renal function was fully restored. Larger, multicenter studies will shed additional insights regarding the optimal use of this treatment modality.
56.6.2 Hyperventilation
The vasoconstrictor effect of hyperventilation on the cerebral arteriolar system has been used in the management of patients with severe TBI for decades. However, contemporary management has veered away from the long-standing “blind” and prophylactic application of hyperventilation in the management of severe TBI. Studies in experimental models have demonstrated that the effects of hyperventilation on CSF pH and arteriolar diameter are short-lived (i.e., vessel caliber returns to baseline in less than 20 hours).99 In addition, chronic hyperventilation produces a loss of metabolic (bicarbonate) buffer in the CSF, putting the cerebral circulation at greater risk because of hypersensitivity of the vasculature to changes in PaCO2. Muizelaar and colleagues100 compared mild versus moderate hyperventilation in adults after severe TBI, showing that moderate prophylactic hyperventilation (PaCO2 = 26 to 27 mm Hg) was not beneficial and was associated with worse outcome at 3 and 6 months. These findings mirror reports demonstrating (1) early posttraumatic hypoperfusion after severe TBI, (2) widening of the arterial–jugular venous oxygen content difference that can be observed early after trauma; and (3) jugular desaturation that can accompany profound hypocarbia. It is important to recognize, however, that although the prophylactic application of hyperventilation can be detrimental, hypoventilation (leading to hypercarbia) is equally worrisome because of potential increases in cerebral blood flow leading to increased cerebral blood volume and intracranial hypertension. In summary, contemporary neurocritical care generally calls for mild hyperventilation to keep cerebral blood flow as normal as possible except in cases of impending herniation (in which hyperventilation to mitigate cerebral ischemia from brainstem compression can be lifesaving). It is likely that future studies demonstrating the feasibility of titrating cerebral blood flow—with serial measurements of CBF from stable xenon CT scan, extrapolated estimates of cerebral blood flow from PbO2 monitoring, or continuous cerebral blood flow measurements from invasive devices—may show a role for hyperventilation in individual children after TBI.
56.6.3 Barbiturates
Barbiturates reduce ICP via a coupled reduction in the cerebral metabolic rate and cerebral blood flow, leading to a decrease in cerebral blood volume and ICP. As with other therapies, there have been no RCTs in children to test the efficacy of barbiturate therapy. Two small RCTs in adult TBI victims failed to demonstrate such an effect, but this should not be interpreted to mean that this therapy should not be considered. Goodman and colleagues101 reported an improvement in brain interstitial concentrations of lactate and glutamate accompanying a reduction of ICP in seven adults treated with barbiturates for refractory intracranial hypertension. Pittman and colleagues demonstrated that 52% of children responded to barbiturate therapy during refractory intracranial hypertension.102 Moreover, Kasoff and colleagues demonstrated that the use of barbiturates in children with TBI caused frequent bouts of hypotension, leading to the recommendation that the use of barbiturates be accompanied by invasive hemodynamic monitoring.77 In addition to cardiovascular monitoring, it is recommended that electroencephalographic monitoring be used to assess the cerebral metabolic response to treatment, with the end point of this therapy generally being burst suppression. It is possible that as the use of hyperventilation wanes in the management of children with refractory intracranial hypertension, alternative therapies such as barbiturates will again be used.
56.6.4 Hypothermia
The theoretical benefits of therapeutic hypothermia have been under study for decades; mechanisms like decreased metabolic demand leading to decreased cerebral blood flow and cerebral blood volume with decreased ICP, alterations in cell death pathways, decreased intracranial hypertension, and others have been postulated. Over time, numerous anecdotal cases and uncontrolled trials have suggested favorable and unfavorable effects. Until very recently, controlled trials of the use of therapeutic hypothermia in human head injury were lacking.
Experimental models of cerebral ischemia and trauma suggest that transient, mild, or moderate hypothermia (32 to 34℃) attenuates excitotoxic neurotransmitter concentrations and the local proinflammatory cytokine response, thereby producing beneficial effects on neuronal recovery and neurologic outcome.103–105 Efficacy for hypothermia was demonstrated in adults after cardiac arrest in 2002, spurring interest in extending this therapy to other brain injuries.106,107 For adults with TBI, hypothermia has had mixed results. Two RCTs (32℃ for 24 or 48 hours) have shown beneficial effects,108,109 with decreased seizure frequency, decreased ICP, and transient improvements in outcomes (especially in subjects with admission GCS scores of 5 to 7). A larger, multicenter trial, however, was unable to reproduce the single-center experience.110
In pediatric TBI, a large RCT was performed to test the efficacy of early hypothermia in improving overall outcome.111 In this most important study, 225 children were randomized to receive hypothermia (32 to 34°C) or normothermia within 8 hours, were maintained at that temperature for 24 hours, and were rewarmed over approximately 16 hours. No beneficial effect was observed in the hypothermia group regarding outcome, with a trend toward increased mortality and decreased functional outcome (Pediatric Cerebral Performance Category Scale score at 6 months) despite hypothermia leading to improvements in ICP. Several potential confounders were noted within the study (the normothermia group received greater amounts of hyperosmolar therapies, both groups had substantial amounts of hyperventilation). Nevertheless, this study has questioned the utility of hypothermia as applied to all children with severe TBI, leading to questions of whether there are specific populations of children that might benefit from hypothermia.
56.7 Surgical Treatment of Elevated Intracranial Pressure: Decompressive Craniectomy
One of the more controversial areas in the management of both adults and children with refractory intracranial hypertension is the use of decompressive craniectomy. Controlled studies of this modality are lacking in adults and children. Described by Cushing in 1905,112 there has been a resurgence of interest in this approach sparked by laboratory studies and several recent case reports suggesting that decompressive craniectomy may result in ICP reduction and a good outcome in selected patients with intracranial hypertension refractory to medical therapy.113 Although some have reported disappointing results,114,115 De Luca and colleagues reviewed 22 cases and reported with guarded optimism that selected patients may benefit.116 Taylor and colleagues117 showed positive results with very early (within 30 hours of injury) decompressive craniectomy for children with intracranial hypertension despite optimal management. The marked reduction in postoperative ICP was deemed the primary neuroprotective outcome in this prospective trial. The common thread in all trials that show positive outcomes are young age (younger than 16 years) and early decompression (within 30 hours of injury). The variable outcomes in the collectively published reports underscore the need for a multicenter RCTs to address the question with a larger sample size. More clarity is needed to determine optimal timing, unilateral versus bilateral craniectomy, and the effect of comorbidities on outcomes. Specific recommendations or guidelines for this procedure cannot be made, and like other second-tier therapies for refractory intracranial hypertension (barbiturates, hypothermia, induced hypertension), decompressive craniectomy is used with varying frequency depending on local experience and the discretion of the management team. A seminal study for decompressive craniectomy in adult TBI victims was recently completed, ultimately randomizing 155 adults with severe diffuse TBI.118 Despite successfully alleviating intracranial hypertension, the surgical group unexpectedly had an increased rate of unfavorable outcomes (odds ratio = 1.84 [1.05 – 3.24], p = 0.03). Technical considerations, patient selection criteria, and differences in medical therapies between groups have all been hypothesized as causes for this curious finding.
56.8 Treatment in the Neurocritical Care Unit
Once the initial resuscitation is completed and evacuable intracranial masses have been addressed, the maintenance of physiologic stability and the recognition and management of intracranial hypertension are the priorities. The injured brain has complex metabolic requirements that are poorly understood. Autoregulation of blood flow may be disturbed, and metabolic demands may be either decreased or increased. It is clear, however, that evidence of neuronal death from cerebral ischemia is a common autopsy finding in patients who die after severe TBI. Maintenance of an adequate CPP is the current therapeutic approach that appears to minimize the risk for the development of secondary ischemia. Assessment of the effect of manipulating CPP on cerebral blood flow or other multimodal markers of cerebral metabolism can provide valuable information and aid in the titration of care. Monitoring of the central venous pressure is essential, and assessment of the cardiac output can be valuable in selected cases. The titration of vasopressor or inotropic support may be needed once adequate filling pressure and hemoglobin are confirmed. In some situations, such as the development of neurogenic pulmonary edema, the optimal titration of cardiopulmonary support can be a formidable challenge and a key determinant of outcome. In addition to the importance of CPP, the selection of an optimal threshold for ICP may play a role. Adult victims of severe TBI with an ICP above 20 mm Hg have a poorer outcome than those without increased ICP. Although a large prospective RCT of patients with and without both ICP monitoring and CPP-targeted management has not been performed, a prospective cohort study by Ghajar and colleagues suggested better outcome in adults monitored and treated with CSF drainage than in those without ICP monitoring.119 Several studies have suggested that optimal outcome is achieved if the neurointensivist responds to even modest levels of intracranial hypertension (i.e., ICP > 15 mm Hg). Further study is needed, particularly in infants and children.
56.8.1 Sedation Analgesia and Neuromuscular Blockade
Sedation and neuromuscular blockade should be used in the setting of intracranial hypertension once appropriate monitoring has been established. Often, in the initial resuscitation, sedation must be carefully titrated. It is difficult to maintain the balance that allows cardiovascular stability, analgesia, and anxiolysis during transport and initial CT yet allows rapid emergence for clinical assessment when a decision regarding surgery or intensive care management may be necessary. Narcotics, benzodiazepines, or small doses of barbiturates are generally recommended for routine use. Recently, for adults, the use of propofol, a nonbarbiturate intravenous anesthetic, has migrated out of the operating theater into the intensive care unit. The utility of propofol in the rapid induction of anesthesia (it works as quickly as thiopental) is surpassed only by its facility in emergence.120 The rapid emergence from sedation with minimal confusion has allowed adult neurointensivists to use propofol preferentially over thiobarbiturates and benzodiazepines for sedation after severe TBI. However, reports in the mid-1990s of an idiosyncratic, lethal propofol infusion syndrome led to the re-evaluation of the role of this general anesthetic in the pediatric intensive care unit.121–124 Subsequently, propofol infusion syndrome in the deaths of several adults has also been reported.125–128 Based on recent recommendations of the FDA, propofol cannot be recommended as a continuous infusion for the sedation of infants and children with severe TBI (www.fda.gov/cder/pediatric/labelchange.htm).
Although neuromuscular blockade has been used commonly in the United States, there has been interest in recent years to more clearly define its indications, duration of therapy, and monitoring. It is currently recommended to use neuromuscular blockade after failure to control ICP with optimized mechanical ventilation, sedation, head position, and temperature control. Once neuromuscular blockade is employed, care must be taken to allow therapeutic monitoring with train-of-four testing as well as daily drug holidays.
To our knowledge, no controlled trial of various sedation regimens has been performed in patients with severe TBI. In contrast, Hsiang and colleagues129 studied 514 adults with severe TBI and suggested that prophylactic neuromuscular blockade was associated with increased length of intensive care unit stay and nosocomial pneumonia. As with most therapies in this setting, careful assessment of indication and meticulous titration of therapy are essential. Finally, intermittent doses of thiopental and/or lidocaine are often needed to blunt excessive rises in ICP secondary to routine patient care maneuvers, such as suctioning.
56.8.2 Cerebrospinal Fluid Drainage
Drainage of the CSF is a direct method to reduce ICP with minimal risks (other than the obvious risk of inserting the ventricular catheter). However, despite widespread use, studies of the effect of CSF drainage on CPP, cerebral blood flow, and neurologic outcome have been limited, particularly in children. Shapiro and Marmarou described the utility of CSF diversion in 22 children in an observational study, reporting that more than 75% of children observed demonstrated decreased ICP and increased brain compliance (measured with the pressure–volume index) after CSF drainage via an externalized drain.79 Fortune and colleagues130 compared the effect of ventriculostomy drainage and mannitol in adults after severe TBI and observed similar effects on cerebral blood flow and ICP. CSF drainage was associated with a greater increase in jugular venous saturation than mannitol administration. CSF can be drained intermittently or continuously in children, with threshold values for drainage determined based on the clinical indication. Interestingly, Shore and colleagues131 suggested that continuous CSF drainage was associated with lower concentrations of a large number of biochemical mediators of secondary injury, such as cytokines, in comparison with intermittent drainage. In addition, a lower ICP was seen with continuous rather than with intermittent drainage. Further study is needed.
56.9 Controversial Issues
56.9.1 Head Position
Head position has been an area of great controversy. Feldman and colleagues132 conducted a prospective randomized study of the effect of head position on ICP, CPP, and cerebral blood flow in 22 patients after severe TBI. Both the ICP and the mean carotid pressure were significantly reduced in the 30-degree position compared with the 0-degree position. There was no change in CPP or flow with this intervention. Thus, in general, raising the head to the 30- degree position reduces ICP without deleterious effects on CPP and is preferred. Head elevation and midline position improve jugular venous drainage and possibly CSF drainage and decrease the contribution of these components to ICP, but the effect overall is not dramatic.
56.9.2 Controlled Hypertension
Another controversial area in management relates to the use of induced hypertension to control refractory intracranial hypertension. Whether pressure autoregulation of the cerebral blood flow is intact or defective, hypotension or an inadequate CPP must be rigorously avoided. If pressure autoregulation is impaired, cerebral blood flow is directly related to CPP, and hypotension directly reduces flow. If pressure autoregulation is intact, as CPP is reduced, reflex cerebral vasodilation occurs (to maintain flow), which increases cerebral blood volume and ICP. Note that this latter phenomenon occurs as CPP is reduced within the autoregulatory range.
Based on measurements of blood flow and jugular venous saturation in adults with severe TBI, an optimal perfusion pressure threshold of 60 to 70 mm Hg is generally observed. Currently, a CPP of 50 to 60 mm Hg is the goal for infants younger than 2 years. Based on the relationship between CPP, vessel diameter, cerebral blood volume, and ICP, in selected patients with refractory intracranial hypertension, the induction of arterial hypertension (CPP increased to between 100 and 140 mm Hg via the infusion of phenylephrine) reduces ICP. However, hypertension reduces ICP only when the pressure autoregulation of cerebral blood flow is intact because it is a hypertension-mediated reduction in vessel caliber that produces the reduction in cerebral blood volume (to maintain a constant flow) and resultant reduction in ICP. In addition, it is unclear what the short- and long-term effects of the applied hypertension are on the development of cerebral edema because the greater hydrostatic pressure applied could exacerbate edema formation. The optimal management of blood pressure after severe TBI requires both extensive monitoring of the involved factors and an in-depth understanding of the mechanisms at work. Induced hypertension is not recommended except as a second-tier therapy and only with careful monitoring. Unfortunately, the use of this intervention is even more complex in the management of infants and children after severe TBI because a single general threshold value of CPP for adequate perfusion is not applicable. Downard and colleagues80 have suggested a minimal value of 40 mm Hg for children. In their report of nearly 200 children with severe TBI, all children with a mean CPP of less than 40 mm Hg died. One would anticipate that this value is directly related to age; however, the relationship has not been defined. Management must be tailored to, and carefully titrated in, each individual patient.
56.9.3 Additional Treatment Issues
Seizures are quite common after TBI and should be aggressively treated to prevent complications related to increased metabolic demand during periods of tenuous cerebral blood flow. However, there is little evidence to suggest that the administration of antiepileptic agents prevents the long-term development of epilepsy. Lewis and colleagues found that the administration of phenytoin led to decreased seizure frequency early after TBI in a small series, ultimately judged to be Level 3 evidence.133
Careful attention must be paid to the serum sodium concentration. An increasing body of literature has developed suggesting the harm that may occur if hyponatremia develops in the acute phase of injury. For this reason, children should generally receive isotonic solutions. If hyponatremia develops, it can be attributed to either syndrome of inappropriate antidiuretic hormone secretion (SIADH) or cerebral salt wasting.134,135 Care should be taken to determine the cause of hyponatremia correctly because the management of SIADH involves fluid restriction, whereas that of cerebral salt wasting involves the administration of isotonic or hypertonic saline.
The provision of adequate calories and protein is essential during the catabolic response to critical illness, and the beneficial effects of early feeding (either enteral or parenteral) in the critically ill or injured patient are well described. In adult TBI victims, increased administration of calories within the first 7 days was associated with improved outcome in a large cohort study from New York State,136 yet similar hypotheses have not been adequately tested in children. In children, an immune-enhanced diet was tested in a small RCT, yet this failed to demonstrate meaningful benefits.137 Moreover, the management of glucose administration has received significant attention in the past several years, with several studies demonstrating a relationship between hyperglycemia and poor outcome.138,139 It is still unclear if treating hyperglycemia would alter this relationship, but it appears obvious at this time that care must be taken to monitor the serum glucose closely after TBI.
Finally, glucocorticoids are not recommended in the treatment of patients with severe TBI unless catecholamine-refractory shock develops. Pituitary stalk injury has been reported after severe TBI and can complicate it.140–142 When corticosteroids are used, a serum cortisol level should be obtained before the treatment dose is administered. This pretreatment level is used to inform the decision to continue therapy.
56.10 Summary
The optimal care of an infant or child with severe TBI requires a multidisciplinary approach in each phase of management. A prompt and vigorous resuscitation including the stabilization and control of ventilation is essential. After the initial evaluation and necessary surgical intervention, multimodal monitoring and the carefully titrated management of intracranial hypertension are recommended to optimize cerebral perfusion, facilitate metabolic homeostasis, and minimize cerebral swelling. Meticulous and optimal neurocritical care management will be the basis for the delivery of future targeted therapies as additional information becomes available on the biochemical aspects of the evolution of secondary neuronal damage and repair.
Pearls
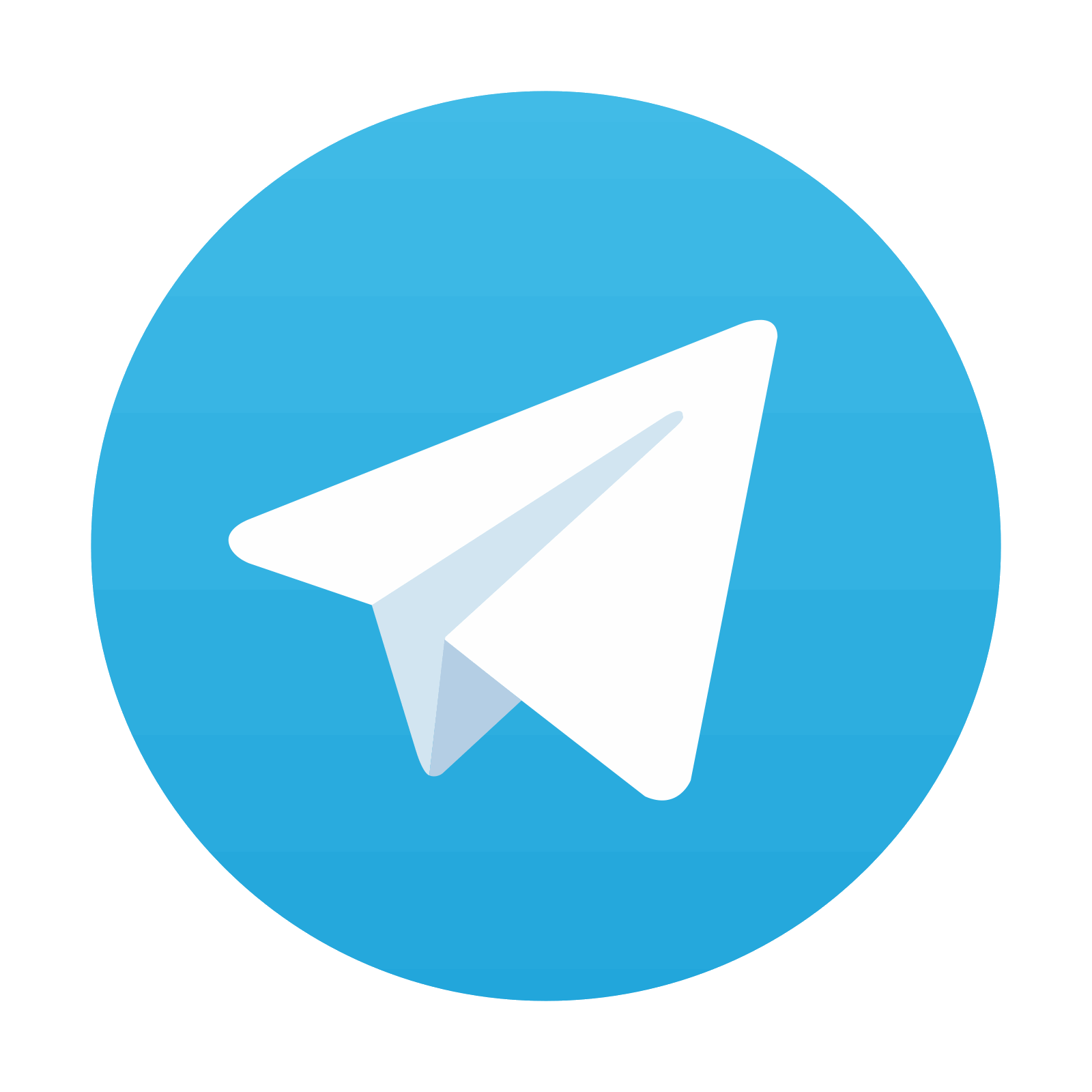
Stay updated, free articles. Join our Telegram channel
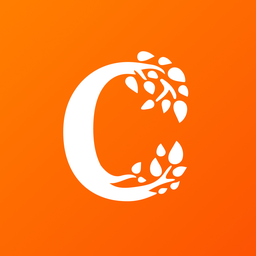
Full access? Get Clinical Tree
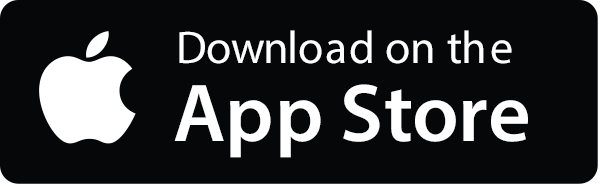
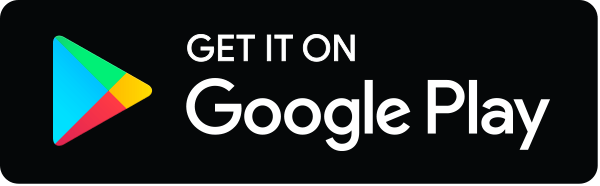