Chapter 4 Vascular Diseases of the Brain
ISCHEMIC CEREBROVASCULAR DISEASE (STROKE)
Clinical Features
Ischemic stroke has been recognized since the work of Hippocrates. Its etiology has been aggressively debated and remains as controversial as its recognition is old. Thromboembolic disease consequent to atherosclerosis is the principal cause of ischemic cerebrovascular disease. Ischemic stroke has been classified by subtypes (Box 4-1) based on a multicenter clinical trial, TOAST, or Trial of ORG 10172 in Acute Stroke Treatment. The most common causes of infarction include large-artery atherosclerosis, cardioembolism, and lacunes. This widely accepted classification scheme allows for assessment of etiology, prognosis, and treatment options. Outcomes differ depending on subtype. Large artery lesions have a higher mortality than lacunes. Recurrent strokes are most common in patients with cardioembolic stroke and have the highest 1-month mortality. Using the TOAST classification, treatment decisions and the outcomes of these treatments can be measured, allowing for both documentation of individual clinical competency and evidence-based assessment of therapeutic efficacy of different treatments and devices. For instance, carotid endarterectomy or stenting is the treatment of choice for large-vessel disease, whereas anticoagulation therapy is most useful in patients with small-vessel disease. Terms such as significant stenosis become rigorous when explicitly defined as greater than 50% to 70%, in particular when this definition has proven prognostic value.
Thromboembolic events are the principal causes of ischemic stroke. Emboli can arise from arterial stenosis and occlusion—atherosclerotic debris and ulceration—in the extremities with coexistent right-to-left shunts, or cardiac sources (a cardiac source of emboli is responsible for 15% to 20% of ischemic strokes). The extent to which narrowing of the arterial lumen contributes to stroke is complex. Even in the absence of severe stenosis the reduction in flow may decrease the ability to “wash out” distal emboli before they produce ischemia (Box 4-2).
Atherosclerosis is common and typically affects multiple extracranial and proximal intracranial vessels or multiple regions within the same vessel. Thirty-five percent of patients over age 50 years have atherosclerotic changes in cervical cerebral arteries, but only one third of these individuals have symptoms of vascular disease. Primary stenosis/occlusion most often results in infarction when there is a preexistent stenosis with either new occlusion or a period of systemic hypotension. Acute extracranial carotid occlusion may produce large areas of infarction involving the deep (ganglionic) and superficial (cortical) middle cerebral artery (MCA) distribution (Fig. 4-1A). In these cases the infarcts are likely the result of large distal emboli associated with the proximal occlusion. The anterior cerebral artery (ACA) territory is typically spared because of collateral supply from the contralateral ACA via the anterior communicating artery (ACoA) of the circle of Willis. Isolated ACA and combined MCA/ACA (“holohemispheric”) (see Fig. 4-1B) infarcts are rare; holohemispheric infarcts are usually fatal. They most often occur in patients with acute myocardial infarction and atrial fibrillation due to the combination of large emboli and poor cardiac output. Alternatively carotid stenosis or occlusion may occur without marked distal embolization, producing “watershed” or border-zone infarction. Vascular watersheds are the distal arterial territories often at borders between two vascular distributions (see Chapter 2). Major border zones are found between the anterior and middle cerebral arteries and the middle and posterior cerebral arteries. Reduction in flow affects these zones to the greatest extent because they are furthest from the heart. Borderzone infarcts occur in the posterior parietal region (MCA/PCA border zone), the frontal lobes (ACA/MCA border zone), and the basal ganglia (Fig. 4-2). These infarcts are often small and may be confused with lacunar infarcts. The key to diagnosis is the presence of multiple infarcts at the interface between different vascular territories and evidence of carotid occlusion or slow flow. Other sites in the brain are selectively jeopardized by hypoxia or hypotension due to increased susceptibility to ischemia from increased metabolic rate and a lack of redundancy of blood supply. These include the hippocampus (Ammon’s horn), globus pallidus, and amygdala (anterior choroidal-posterior cerebral watershed), cerebellum, and occipital lobes, in that order.
Interest in the detection and treatment of extracranial carotid artery disease has been heightened by the results of two large trials for the treatment of symptomatic and asymptomatic patients. The North American Symptomatic Carotid Endarterectomy Trial (NASCET) and the Asymptomatic Carotid Atherosclerosis Study (ACAS) confirmed the benefit of carotid endarterectomy in patients with high-grade carotid stenosis (>60% or more). The widespread availability of noninvasive vascular imaging (ultrasound, MRA, and CTA) and the introduction of stent devices for the carotid artery have resulted in a dramatic increase in the number of individuals being treated for carotid stenosis. The assessment of degree of stenosis is complicated by the existence of various methods for measuring stenosis. NASCET uses the ratio of the stenosis to the “normal lumen” distal to the stenosis, whereas ACAS and the European studies use the degree of stenosis relative to the estimated normal lumen at the same site. Each method has its limitations. The NASCET criteria can lead to underestimation of stenosis when the distal lumen narrows as a result of the severe proximal stenosis. The ACAS method is problematic because the observer must extrapolate what is thought to be the true lumen (Fig. 4-3). A more promising technique may be to simply measure the cross-sectional area of the stenosis. Recent studies have shown that when the vessel lumen is less than 1.3 mm the stenosis is at least 70%.
Intracranial embolic occlusion most commonly produces infarction in the midsection (posterior frontal, anterior parietal, and superior temporal) of the MCA distribution (see Fig. 4-1A). Emboli entering the carotid artery preferentially lodge in these MCA branches. Pure ACA embolic infarcts are rare. Isolated ACA infarcts (see Fig. 4-1B) typically occur as a result of intrinsic arterial disease and occlusion (e.g., diabetes, hypertension, vasospasm, and vasculitis) or from severe subfalcine herniation rather than emboli. The location and extent of the infarct will be determined by the site of embolic occlusion and extent and location of collateral supply to the brain distal to the occlusion. Occlusion of the distal carotid bifurcation and proximal MCA and ACA vessels (T occlusions) may result in infarction of the cortical (superficial) and ganglionic (deep) portions of the MCA territory. If there is good cortical collateral supply, the infarct may be confined (at least initially) to the basal ganglia and insula, in part due to lenticulostriate branch obstructions. Embolic infarcts in the vertebral basilar system may affect single or multiple vessels. Complete basilar occlusion produces cerebellar and brain stem infarcts and variable bilateral infarction of the inferior medial temporal and occipital lobes and posterior thalami, whereas basilar tip occlusions spare the posterior fossa structures (see Fig. 4-1D and E). The extent of posterior cerebral artery involvement depends on the status of the posterior communicating arteries (PCoA). Focal occlusion of the distal vertebral artery produces infarcts in the distribution of the posterior inferior cerebellar artery (PICA), leading to infarcts in the inferior cerebellum and lateral medulla (Wallenberg syndrome) (see Fig. 4-1C).
Lacunar infarcts are small lesions produced by occlusion of deep perforating arteries. Lacune is a venerable pathologic term indicating a fluid-filled hole in the brain (Fig. 4-4). The TOAST criteria define lacunes based on size, location, and etiology rather than gross pathology. I used to rail against the use of the term lacunar infarct on CT reports because one cannot tell on CT whether the lesion is fluid filled or just gliotic. Although this distinction can be made on fluid-attenuated inversion recovery (FLAIR) MRI, the point is moot because lacune now has a formal meaning; by definition, it is an infarct of less than 15 mm. Lacunes have a predilection for the basal ganglia, internal capsule, pons, or corona radiata (see Fig. 4-3A and B). Occlusion of brain stem perforating arteries produces distinctive infarcts that are paramidline, unilateral, and tubular in appearance on axial imaging, reflecting the location and course of the pontine perforating arteries (see Fig. 4-4C). Although these infarcts were originally thought to arise from small-vessel atherosclerosis and lipohyalinosis associated with hypertension, many other causes have been proposed, including emboli, hypercoagulable states, vasospasm, and small intracerebral hemorrhages.
IMAGING TOOLS
Brain
where D is the diffusion coefficient, S0 is the signal intensity of the unweighted image, S(b) is the signal intensity of the images for various b values, and the b value is specific for the particular pulse sequence used to measure diffusion. b is a function of the diffusion gradient strength, the duration of the diffusion gradient pulse, and the time of the diffusion measurement. The b value determines the degree to which an image is sensitive to diffusion (the higher the b value the more diffusion weighted an image becomes). The ADC can be calculated by using images with varied gradient strengths (different b values). At a minimum ADC can be calculated if there are at least two b values, one of which must be set to approximately 0; that is, with no diffusion weighting. In clinical practice two b values are generally used; however, four or more b values can be measured to improve accuracy of measurement. Commonly used values include a b value of 800 to 1,200 sec/mm2, with time to echo (TE) of 90 to 120 msec.
Vessels
Angiography
The advent of 3 Tesla (3T) MR scanners has produced a dramatic improvement in TOF MRA (Fig. 4-5). This is related in part to increased signal-to-noise ratio; however, a more important cause is increased T1 of normal tissues when imaged at 3T. Because the T1 is longer it is easier to suppress background signal at 3T, resulting in a marked improvement in visualization of flowing intravascular protons. This effect is most notable on intracranial MRA. At 1.5T visualization of second-order intracranial branches (e.g., intrasylvian MCA branches) is limited; therefore, detection of distal occlusions, vasculopathy, and arterial spasm is not reliable. At 3T these vessels and even smaller arteries (e.g., lenticulostriate arteries) are well visualized in almost all cases (see Fig. 4-5). Therefore, it is preferable to perform MRA studies on 3T scanners.
Contrast-enhanced MRA uses paramagnetic contrast enhancement in association with 3D TOF imaging. This method has many advantages over the noncontrast approach. Like CTA (see below) the technique visualizes contrast within vessels and is not dependent on flow. The result is a rapidly acquired (<30 sec) high-resolution image of the extracranial and proximal intracranial vessels with typical coverage from the aortic arch to the circle of Willis (Fig. 4-6). Timing is critical because enhancement of veins confounds the ability to demonstrate arterial anatomy and the sequence is typically triggered with MR fluoroscopic techniques. This technique offers excellent visualization of the aortic arch and proximal cervical vessels. These structures are not seen on routine TOF MRA and may be difficult to visualize on CTA. Because it is not dependent on flow and not affected by turbulence it is superior to noncontrast MRA for evaluation of carotid bifurcations and cervical and intracranial vertebrobasilar systems. It also can decrease ambiguity in cases with flow reversal such as subclavian steal (Fig. 4-7). CEMRA is not used for the routine assessment of intracranial vessels because of problems produced by venous contamination and enhancing normal (sinus mucosa) and pathologic tissues (e.g., brain tumors).
Computed tomographic angiography (Fig. 4-8) has emerged as an alternative to MRA for imaging both the extracranial and intracranial blood vessels with the development of multirow detector scanners. Current 16- to 64-row scanners can provide excellent visualization of extracranial and intracranial vessels without venous contamination (assuming accurate timing of contrast bolus injection, which is frequently a BIG assumption). New 320-row detector scanners can acquire data from the entire brain simultaneously and therefore, with multiple acquisitions, produce time-resolved angiographic studies that mimic catheter angiography in their appearance. CTA requires the placement of a catheter, usually in the antecubital vein, with rapid injection of approximately 50 to 125 mL of iodinated contrast material. After a short delay following contrast injection, imaging commences and a 3D data set is acquired. CT advances have resulted in thinner images, improving resolution. Computer postprocessing is necessary for MIP images and for excluding the bony base of the skull structures. In the past postprocessing was a relatively time-consuming, labor-intensive task requiring knowledge of anatomy and the ability to use 3D workstations. As 3D workstations have been improved, the task of reconstructing CTA studies has become easier and can now be done (at least preliminarily) by technologists at the CT scanner. It has gained immense popularity in the workup of hyperacute infarction when used in combination with CT and CT perfusion because of availability and ease and speed of data acquisition. It is superior to MRA for detecting and characterizing smaller aneurysms at the cost of radiation and iodinated contrast dye. CT and CTA are typically performed at initial presentation of suspected aneurysmal SAH (aSAH) on the ED scanner because all data can be collected in less than 2 minutes.
Computed tomography angiography has several advantages when compared to MRA. Because the images are not motion-sensitive, CTA allows for accurate assessment of extracranial stenosis (see Fig. 4-8C and D). Workstations often have software that allows for measurement of cross-sectional areas at multiple sites. Calcification does not cause the same artifacts that are seen on MR, and extremely slow flow and tandem lesions are more reliably detected on CTA than MRA. Intracranial embolic occlusion is more easily seen, and focal clot within proximal intracranial vessels may be directly visualized (see Fig. 4-8E). CTA has better spatial resolution than MRA; therefore, identification of aneurysm morphology (including overall size and neck morphology) and relationship to adjacent and parent vessels is better (see Fig. 4-8F). The superb quality of CTA has prompted many neurosurgeons to operate directly on the basis of CTA findings, reserving catheter angiography for those cases where CTA findings are inconclusive or when endovascular treatment is to be performed. Interestingly, over the past few years there has been a movement back to performing catheter angiography even when surgery is the treatment of choice. This may reflect improvements in digital sub- traction conventional angiography equipment with ability to perform rotational (3D) images during arterial contrast injections. Although MRA is more accurate for the assessment of aneurysms treated with endovascular coils, CTA has proven to be more accurate for the assessment of aneurysms treated with surgical clipping.
The limitations of CTA include: (1) risks of intravenous iodinated contrast injection; (2) exposure to radiation; (3) obscuration of vessels at the base of the skull due to bone and contrast in the cavernous sinus; (5) obscuration of aneurysms by extensive SAH; (6) extensive atherosclerotic calcifications in the walls of the vessels; (7) atherosclerotic calcifications and normal osseous structures, such as the anterior clinoid process, obscuring the underlying vessel and less frequently mimicking the appearance of an aneurysm on CTA surface renditions (see Fig. 4-8G and H); (8) the operator-dependent nature of the 3D reconstruction process. Calcification can be a problem with CTA, MRA, and even catheter angiography. With moderate calcification CTA is superior to MRA for assessing degree of stenosis, but with heavy calcification the lumen may be obscured on CTA but visible on MRA.
Arterial catheter angiography (Fig. 4-9) is the definitive imaging modality for vascular lesions of the brain and great vessels of the neck but has been relegated to a secondary role in the diagnosis of stroke. Patients are referred for angiography for the following reasons: (1) if the MRA, CTA, or carotid ultrasound are equivo- cal; (2) if MRA is contraindicated (e.g., in patients with pacemakers); (3) if cardiac output is too low to produce a diagnostic CTA; (4) to evaluate complex aneurysms or vascular malformations responsible for an intracranial hemorrhage; and (5) for the evalua-tion of vasculitis. The advent of rotational 3D digital subtraction angiography has made it possible to combine the advantages of selective arterial injection of contrast with the 3D imaging intrinsic to CTA.
In individuals with acute or chronic ischemic disease angiography is used in selective cases, in particular if endovascular intervention is contemplated. It is an excellent albeit invasive method for determining whether a lesion is hemodynamically significant in the carotid circulation (Box 4-3). Assessment of collateral circulation distal to a stenosis or occlusion is most easily determined with catheter angiography, where serial images show the presence, source, and extent of collateral supply to the brain.
Box 4-3 Angiographic Findings in Hemodynamically Significant Lesions of the Extracranial Carotid Arteries
Detection of ulcerated plaques is more accurate with catheter than noninvasive angiography. However, on all types of angiographic examinations it is difficult to distinguish ulceration from irregularity. The most reliable angiographic sign is the penetrating niche, but depression between adjacent plaques and intraplaque hemorrhage may produce a similar appearance (see Fig. 4-9). Luminal bulging secondary to destruction of the media with an intact intima can also appear as an ulcer. One should appreciate that the association of ulcer and stroke is also controversial. Many asymptomatic plaques are ulcerated and many symptomatic plaques are not. Generally, however, ulceration is frequently found on the symptomatic side in association with significant stenosis. High-resolution surface coil-enhanced MR imaging is an excellent way to evaluate ulcerated plaque but requires hands-on study to optimize planes of section and flow suppression. The best approach presently is for the radiologist to describe the plaque as smooth or irregular, and if an undermined niche is present, the term ulceration can be used. It is in the province of the physician caring for the patient to base therapy on the severity of findings and on the patient’s symptoms. No studies have documented any greater risk of angiography during an acute stroke. The vascular supply to the symptomatic region should be the first order of business. What is the current role of angiography in hyperacute stroke? It is primarily used in an interventional mode for thrombolysis and stenting.
Perfusion
Cerebral blood flow is determined by the ratio of cerebral blood volume (CBV) divided by the mean transit time (MTT). The CBF of the normal brain ranges between 45 and 110 mL/100 g of tissue/min. Cerebral oligemia (about 20 to 40 mL/100 g/min) is defined as underperfused asymptomatic region of brain that will recover spontaneously, whereas an ischemic hypoperfused brain is symptomatic and at risk to develop irreversible infarct without revascularization. The ischemic threshold identified in animal experiments when there is cessation of action potential generation occurs around 20 mL/100 g/min and the infarction threshold, associated with irreversible neuronal damage, is at approximately 10 mL/100 g/min. Therefore, ultimately, it is CBF that determines whether tissue will live or die, but changes in MTT and CBV reflect the pathophysiologic processes that precede and then determine when CBF decreases to nonviable levels. The initial event is an increase in MTT due to an occlusion or stenosis. MTT will be determined by the site of occlusion or stenosis and the presence and type of collateral supply to the affected brain. The autoregulatory response of the brain is vasodilation of the vascular bed distal to the occlusion or stenosis and increased oxygen extraction from the blood. Vasodilation increases CBV; therefore, initially CBF is maintained or at least does not decrease to the level where neuronal death occurs. However, once maximal vasodilation is achieved any further increases in MTT (due to progressive occlusion, new embolization, or decrease in systemic blood pressure) will result in decrease in central perfusion pressure, collapse of the vascular bed, and decrease in CBV and consequent decrease in CBF.
Perfusion imaging can be performed in a number of ways, but by far the most common technique in clinical practice involves an intravenous injection of contrast material that does not traverse the blood-brain barrier. Rapid sequential imaging (images every 15 sec) of all or part of the brain allows the visualization of the effect of the contrast agent as it traverses the vascular system. This “bolus tracking” technique is used for both MR perfusion (MRP) and CT perfusion (CTP). In CTP the density of the brain increases while the iodinated contrast agent passes the vascular supply; with MRP the intensity of the brain decreases because the paramagnetic gadolinium agent causes T2-shortening (dynamic susceptibility imaging). In both cases one obtains direct measurement of CBV (it is the area under the curve of the density/intensity change). The time that it takes the contrast to traverse the brain is the MTT; therefore, the CBF can be calculated using the central volume principle. However, to precisely measure CBV and MTT it is necessary to eliminate the contribution of contrast within small arterioles and venules. This requires mathematical “deconvolution” of the data. This is easy with CTA, where data from the arterial input and venous output (obtained by measuring the changes in density within large arteries such as the anterior cerebral arteries and large veins such as the superior sagittal sinus) can be obtained. With MR, this is more difficult because of the contribution of flow effects within large vessels. Therefore, the values obtained from CTA are precise mathematical measures of the three perfusion parameters, whereas those obtained with MR perfusion are relative values (e.g., rCBV, rCBF, rMTT). With both CTA and MRA parametric MTT, CBV, and CBF maps are generated and evaluated qualitatively. Measurement of absolute values is only possible with CTP. The parametric maps provide somewhat different information, and each has its advantages and limitations. Because the initial event in an infarct is increase in MTT, the MTT maps are the most sensitive to early ischemic changes, but because not all areas of elevated MTT go on to infarction MTT maps tend to overestimate final infarct volume. What measure best correlates with the size of the final infarct? It depends on many factors, including what literature you read. CBV maps appear to have the best correlation with the ultimate infarct volume. However, this is controversial, with some reports indicating that rCBV underestimated final infarct volume whereas rCBF overestimates it. Such differences may, in part, be related to when the measurement is made (12 hours versus 24 hours). Perfusion imaging is critical to determining whether or not there is salvageable brain that can be protected by use of intravenous or intra-arterial thrombolytic therapy (tPA), medical therapy, or mechanical clot removal devices (MERCI and Penumbra). All of these treatments are associated with an increased risk of intracranial hemorrhage; therefore, treatment should be reserved for individuals who can benefit from recanalization. Individuals in whom the area of infarction corresponds to the area of abnormal perfusion should not be treated regardless of other factors (time from onset of symptoms, extent of infarcted brain) since there is no brain to protect. On the other hand in patients where brain at risk is greater than the already infarcted brain by more than 20%, treatment is likely to result in improved outcome. The brain at risk is described as the ischemic penumbra. On MR, the penumbra is the brain tissue surrounding the core diffusion-“positive” (hyperintense) infarcted brain that has normal diffusion but abnormal relative perfusion (diffusion/perfusion mismatch). On CT there is no easy direct way to measure the extent of the already infarcted brain; therefore, it is necessary to use quantitative measures of perfusion to define the predicted infarcted brain (<10 mL/100 g/min) and the penumbra (10–30 mL/100 g/min) (Figs. 4-10 and 4-11).
< div class='tao-gold-member'>
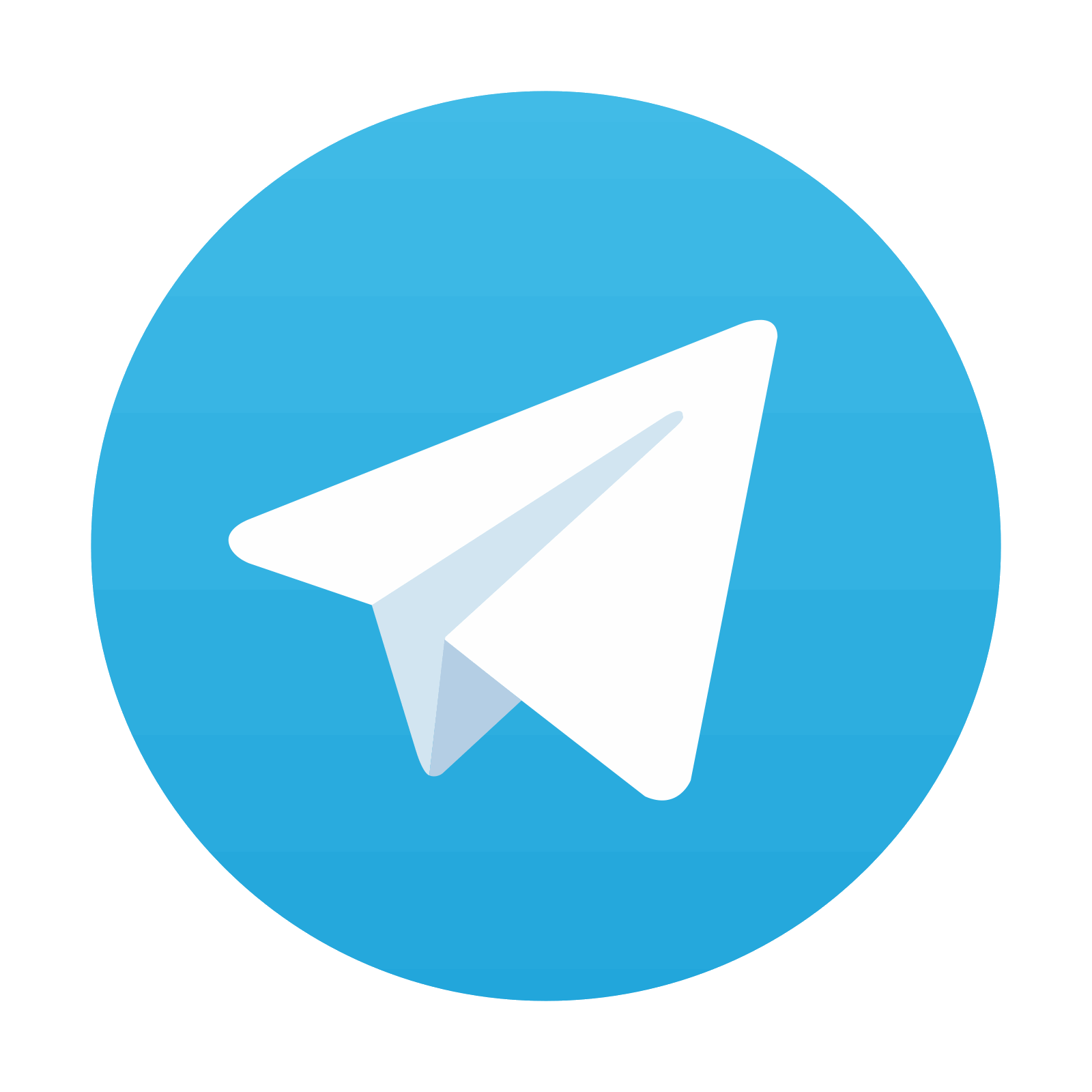
Stay updated, free articles. Join our Telegram channel
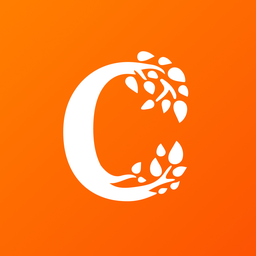
Full access? Get Clinical Tree
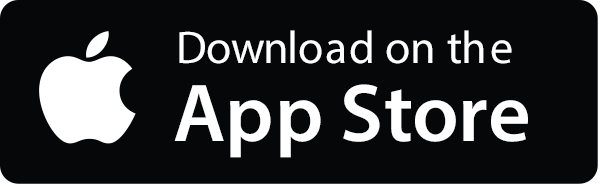
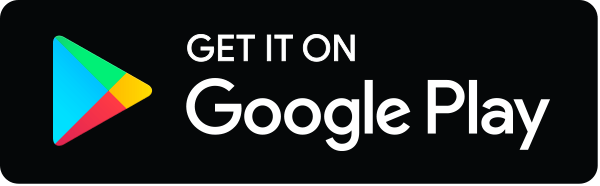