Proprioception
This chapter is concerned with those mechanoreceptors that provide us with information about ourselves: about the positions and movements of our limbs, the forces generated by our muscles, and our attitude and motion relative to the earth. The brain mostly uses this information to help control movement; consequently a discussion of how proprioception is used to control muscle length is left until Chapter 10, and its use in maintaining an upright posture to Chapter 11.
Muscle proprioceptors
Two distinct kinds of proprioceptors are found in voluntary muscles, specialized for providing information about two quite different things: muscle spindles that respond to muscle length and rate of change of length, and Golgi tendon organs that signal muscle tension or force. Both are essentially stretch receptors: their difference in function comes about because of their different situation in the muscle as a whole. Whereas the spindles are in parallel with the main contractile elements in the muscle, so that their stretching is simply a measure of the degree of stretch x of the muscle itself, the tendon organs are situated in the muscle tendons, in series with the contractile elements and the load, so that their stretch is proportional to the tension T exerted by the muscle. Consequently, when a muscle contracts the tendon organs are stimulated by the extra tension, but the activity of the sensory elements in the spindles is reduced as they are less stretched.
![]() |
Spindles
Muscle spindles are found in practically all the striated muscles of the body, but are greatly outnumbered by the striated muscle fibres themselves: in cat soleus, there is only one spindle for every 500 or so ordinary fibres. Each consists of a fluid-filled spindle-shaped capsule some 2-4 mm long and a few hundred micrometres in diameter, whose ends are attached to the exterior sheaths of neighbouring muscle fibres. Inside is a small number of modified muscle fibres called intrafusal fibres (the ‘fus-’ root means ‘spindle’), each having contractile ends, and a region in the middle that is not contractile but contains the nuclei. Though contractile, their direct contribution to muscle tension is negligible: this is done by the ordinary extrafusal fibres, with a completely separate motor innervation.
![]() |
Two main types of intrafusal fibre are found, differing in the way in which these nuclei are distributed. Nuclear chain fibres are thinner, and their nuclei are lined up in a row along the central portion like peas in a pod; nuclear bag
fibres have a pronounced bulge in the middle in which the nuclei are bunched together. A typical spindle has some half-dozen intrafusal fibres, the nuclear chain fibres generally being in the majority. Two kinds of afferent or sensory fibre innervate the spindle: the larger, primary fibres, belonging to group Ia, send branches to the central portions of both types of fibre and have annulospiral endings; the smaller secondary fibres are of group II and terminate partly as annulospiral and partly as flowerspray endings mainly on the nuclear chain fibres, more peripherally than the Ia endings.
fibres have a pronounced bulge in the middle in which the nuclei are bunched together. A typical spindle has some half-dozen intrafusal fibres, the nuclear chain fibres generally being in the majority. Two kinds of afferent or sensory fibre innervate the spindle: the larger, primary fibres, belonging to group Ia, send branches to the central portions of both types of fibre and have annulospiral endings; the smaller secondary fibres are of group II and terminate partly as annulospiral and partly as flowerspray endings mainly on the nuclear chain fibres, more peripherally than the Ia endings.
These two kinds of nerve fibre respond very differently to muscle stretch. The secondary fibres are in a sense simpler: their signals are more or less directly proportional to the degree of stretch of the spindle at any moment. Whether the muscle is suddenly stretched to a new length, stretched more slowly, made to shorten, or alternately stretched and relaxed in a sinusoidal manner, their frequency of firing mirrors quite accurately the instantaneous value of the muscle length, as can be seen in the idealized responses below. They are therefore essentially non-adapting or static. The Ia fibres, by contrast, are dynamic and show very pronounced adaptation. During a sudden stretch they fire maximally during the period of stretching and only at a reduced rate when the muscle is held at its new length; during a slow stretch they again respond most during the movement, at a frequency nearly proportional to the rate of stretch; and during sinusoidal stretching their maximum firing is not at the moment of maximum stretch but near the point of maximum rate of change of stretch. In other words, they respond partly in proportion to muscle length but mostly in proportion to its rate of change, or velocity: this means that they are in effect predicting the future length of the muscle, an important feature in providing feedback to the motor control system (see p. 193 and p. 208).
![]() |
Now we saw in Chapter 3 that adaptation in sensory receptors can in general be due to two distinct processes: there may be energy filtering, in which static information is wholly or partly thrown away before it even reaches the transducer element itself; or there may be membrane adaptation, in which even a steady conductance at the ending results in a fall-off in firing frequency. Whereas in the Pacinian corpuscle both of these mechanisms contribute almost equally to the adaptation that is observed, in the case of the muscle spindle it appears that nearly all is of the energy-filtering kind, and not very different from what is produced by the concentric lamellae of the Pacinian corpuscle. The contractile portions of the intrafusal fibres behave as if they were very much more viscous than the central portion (this is particularly true of the nuclear-bag fibres), so that the mechanical properties of the fibres as a whole may be represented by a mechanical model like the one below. In a brief stretch, there is no time for the viscous elements to lengthen, and the stretch is entirely taken up by the central region, where the annulospiral endings are. But if the stretch is maintained, the viscous elements gradually yield, releasing the strain on the middle portion, and causing the frequency of firing to drop. The difference between the non-adapting properties of the secondary fibres and the marked adaptation of the primaries seems partly to be due to the fact that the former go only to chain fibres, which are less viscous, and also to the fact that they innervate more peripheral parts of them.
![]() |
Besides the two kinds of afferent fibre, spindles also receive motor innervation from the γ fibres, belonging to group Aγ and around 6 µm in diameter. There are two types of fusimotor fibre, called γs and γd – static and dynamic – and they innervate, respectively, the nuclear chain (mostly) and nuclear bag fibres, causing contraction of the peripheral regions.
For any given muscle length, such a contraction must of course stretch the sensory elements and thus increase the firing of the afferent fibres, and in fact the effect of γ stimulation is in general much the same as if an extra stretch had been applied to the muscle as a whole, though they may also increase the sensitivity of the endings to stretch, by altering the elasticity of the intrafusal fibres and thus changing the proportion of the stretch that is experienced by the stretch receptors themselves. The graphs overleaf show how the firing frequency of stretch receptor afferents (from the eye muscles of a goat) responds to different degrees of stretch, when the corresponding γ fibres are also stimulated at different rates: the interaction between external stretch and the internal stretch produced by the γ activation can be clearly seen. The static and dynamic γ fibres produce slightly different effects on primary and secondary afferent responses, as would be expected from their differing distribution to the energy-filtering bag and
non-adapting chain fibres. Dynamic γ fibres increase the sensitivity of group Ia fibres but have no effect on group II fibres, whereas the static ones increase the sensitivity of both the secondaries and primaries to static stretch, and actually decrease the primary sensitivity to rate of stretch (the mechanism is unclear). Thus the central nervous system can, through the γ efferents, control not just the sensitivity of the spindle afferents, but also in a sense their adaptational properties. Consideration of the way in which γ control is actually used by the motor system is postponed to Chapter 10.

non-adapting chain fibres. Dynamic γ fibres increase the sensitivity of group Ia fibres but have no effect on group II fibres, whereas the static ones increase the sensitivity of both the secondaries and primaries to static stretch, and actually decrease the primary sensitivity to rate of stretch (the mechanism is unclear). Thus the central nervous system can, through the γ efferents, control not just the sensitivity of the spindle afferents, but also in a sense their adaptational properties. Consideration of the way in which γ control is actually used by the motor system is postponed to Chapter 10.
![]() |
Golgi tendon organs
The Golgi tendon organs have received much less attention from experimenters. In appearance they are very similar to the Ruffini organs in the skin (Chapter 4) and, like them, appear to respond to tension in the fibres with which they are associated in the tendon; they are innervated by afferents of group Ib. At one time their importance was underestimated because they seemed to have such high thresholds: large tensions had to be applied to the tendon as a whole before they could be induced to fire. But it is now clear that this was because in these circumstances the total tension applied is in effect shared out among the tendinous fascicles, so that each tendon organ feels only a small fraction of it. They respond in fact very briskly to the modest, active tensions generated by the actual muscle fibres to which they are joined: the contraction of less than a dozen motor units can be enough to generate significant activity in a tendon organ. Because they register tension rather than muscle length, during active movements their discharge generally has a reciprocal relationship to that of the muscle spindles: extrafusal activity simultaneously increases the tension in the tendons and decreases muscle length; but during passive movements, both kinds of response are normally in step with one another. Like many other mechanoreceptors, they respond partly to change, in this case changing load or tension.
The function of the tendon organs is not entirely clear. Information about the forces being generated during movement is certainly vitally important to the central controlling mechanisms, particularly in sensing load and in learning to generate appropriate commands to achieve particular movements: this is discussed in Chapter 10 and Chapter 12. The clasp-knife reflex has an obvious protective function, in helping to ensure that the tension in a tendon does not reach the point of rupture; it has also been suggested that Golgi tendon organs ensure that each group of motor units contributes equally to the development of total force by the muscle as a whole.
Central projections
The central pathways of afferents from both muscle spindles and tendon organs are quite similar. Fibres enter the dorsal roots in the usual way, and branches of the majority synapse in a spinal nucleus extending roughly from L3 to T1 called Clarke’s column (or dorsal nucleus) with neurons whose fibres ascend in the homolateral posterior spinocerebellar tract to an extremely important region in the control of movement, the cerebellum. However, since Clarke’s column fades out above T1 or so, more rostral afferents turn upwards and ascend to the accessory cuneate nucleus of the medulla, from which fibres run in the cuneocerebellar tract; they carry information from the forelimbs, which are not represented in the dorsal pathway. Another route (not shown in the figure) by which both cutaneous and proprioceptive information may reach the cerebellum is via the spino-olivary tract to the inferior olive, which in turn projects through climbing fibres to the cerebellar cortex (see Chapter 12). Apart from ascending to the cerebellum, branches of fibres from muscle proprioceptors are involved in various reflex mechanisms
within the spinal cord, notably the stretch reflex (which in its simplest form consists of a monosynaptic excitation of a motor neuron by a Ia afferent) and the clasp-knife reflex: these are discussed in Chapter 10. Muscle proprioceptors also project to the cerebral cortex, via the ventral posterior thalamus.
within the spinal cord, notably the stretch reflex (which in its simplest form consists of a monosynaptic excitation of a motor neuron by a Ia afferent) and the clasp-knife reflex: these are discussed in Chapter 10. Muscle proprioceptors also project to the cerebral cortex, via the ventral posterior thalamus.

![]() |
Joint receptors
Another important source of information about limb position and movement comes from the mechanoreceptors that are found in the ligaments and capsules of joints. They are of a variety of morphological types, some very similar or identical to those found in the skin. Thus Pacinian corpuscles and Golgi-like endings are found with large axons (group I), Ruffini endings (II), and also small nerve fibres with unencapsulated endings. Some show complete adaptation and are thus more sensitive to rate of change, but most show incomplete adaptation and thus signal limb position as well, as can be seen in the recordings below, from afferents from a cat kneejoint as the knee is bent through a fixed angle at different rates. The patterns of response are complicated to a certain extent by the fact that few receptors are able to respond over the whole range of movement that a joint is capable of: their ‘excitatory angle’ is typically less than half the entire possible range, thus increasing sensitivity to changes in position within that range. This means that information about the position of a limb is partly coded by frequency of firing, but also by which neurons are firing. Although in some joints, most of the afferent fibres fire preferentially at extremes of joint position and are presumably intended to give warning that the joint is about to become dislocated, nevertheless there appear to be sufficient fibres responding at mid-range positions to provide adequate proprioceptive information; in other joints the majority are mid-range anyway. Afferent information from joints follows the same route as that from Pacinian and other corpuscles in the skin (see p. 81): fibres ascend in the ipsilateral posterior columns, relay in the cuneate and gracile nuclei, cross and proceed via the medial lemniscus to the ventral posterolateral thalamus and thence to the somatosensory cortex; some also contribute to the spinocerebellar pathways.
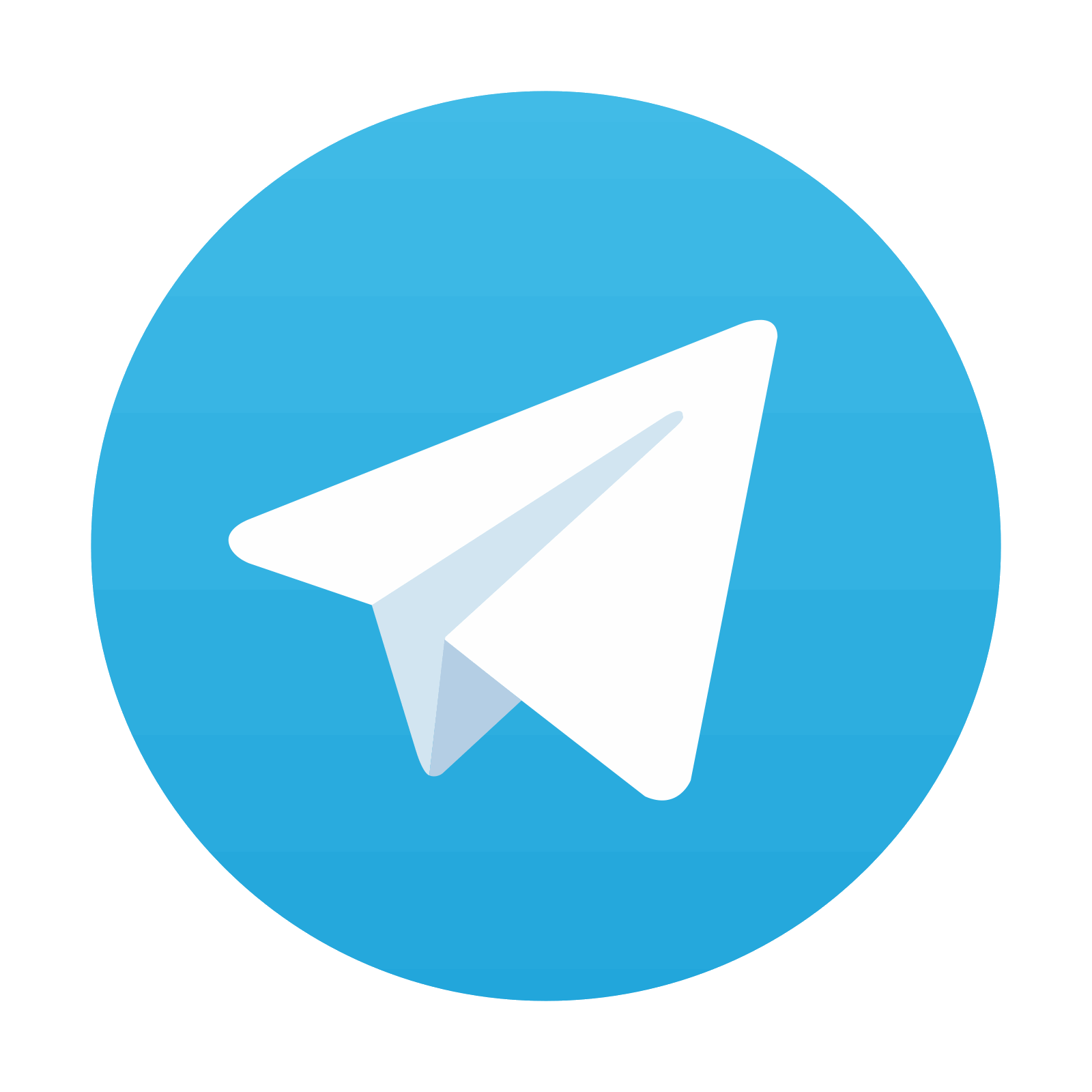
Stay updated, free articles. Join our Telegram channel
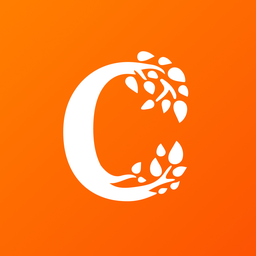
Full access? Get Clinical Tree
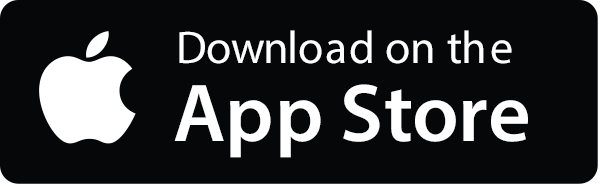
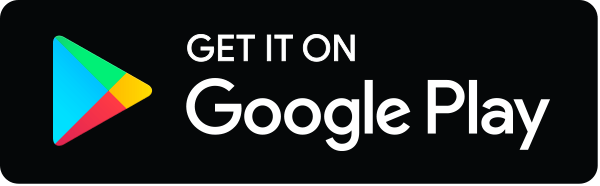