CHAPTER 71 Neuroanatomical Systems Relevant to Neuropsychiatric Disorders
OVERVIEW
In this chapter, we will provide an overview of the anatomy and function of brain systems of particular relevance to the pathophysiology of psychiatric disorders—those subserving emotional and cognitive functions that are crucial to the organization of responses to the environment that promote the well-being and survival of the individual. Thus, we have focused on three neuroanatomical systems: the prefrontal cortex (PFC) (which mediates flexible control of goal-directed behaviors and regulates affective responses); the basal ganglia (BG) (which collect and integrate a wide range of sensory and affective information about the environment to direct the function of the PFC and initiate adaptive actions); and the medial temporal lobe (MTL) (which encodes, stores, and retrieves specific memories, as well as labels the emotional value of objects and situations, so that moment-to-moment interactions with the world optimally reflect knowledge gained from experience). These three systems interact with one another and with other regions of the brain to produce beliefs, decisions, emotional reactions, enduring mood states, and actions. We will also describe some of the evidence for abnormalities in these three systems in psychiatric disorders, emphasizing that it is likely that most psychiatric illnesses arise from abnormalities in the func -tion of specific networks of brain regions that include several regions that project to one another to serve a specific operation of the brain. For example, recent studies have found evidence for abnormal physiological interactions between the PFC and BG in addiction, schizophrenia, depression, and obsessive-compulsive disorder (OCD), and in the interactions of the PFC and MTL in anxiety disorders and schizophrenia. The identification of the unique pattern of functional change that characterizes each disorder is currently the subject of active investigation.
Before turning to the details of the organization of the PFC, BG, and MTL, several overall features of brain organization should be mentioned briefly. The brain tends to process incoming information from the environment in a predominantly unidirectional manner, with feedback given to previous stages of processing at many points along this input pathway. Thus, in general, sensory information is collected by the peripheral nervous system and the cranial nerves and then sent to the thalamus, which then sends information to separate territories of unimodal primary sensory cortex (i.e., visual, auditory, or somatosensory). Primary sensory information is then processed further in unimodal and polymodal association cortices, where this perceptual information is integrated and reconciled with perceptual expectations and information from other sensory modalities (in the polymodal areas), under the modulatory influence of selective attention. Thus, cortical processing proceeds from a detailed picture of a large portion of the external environment to an increasingly abstracted representation of the sensory data, such that only specific information that is required to serve the immediate needs of the individual is readily accessible. Higher-level cognitive operations in the polymodal association cortex in humans tend to be lateralized, with linguistic and computational processing occurring to a greater extent in the left hemisphere, while spatial, holistic types of processing occurring predominantly in the right hemisphere. There is evidence that emotional processing (both the evaluation of the emotional meaning of a stimulus and the expression of an emotional response), particularly of negatively valenced emotions (such as sadness), occurs preferentially in the right hemisphere,1,2 perhaps because emotional appraisal mechanisms are gestalt-like and holistic in nature.
Along this processing stream, the increasingly abstract representation of the outer world is integrated with sensory information sent from the internal milieu (e.g., “Am I hungry, tired, in pain, or relaxed?”); memories of past experience (e.g., “Have I seen this before?” “Who is this person?” or “Where am I?”); and current goals (e.g., “What am I trying to do now?” or “What do I want?”). This internally generated information is collected by a loosely defined group of brain regions called the limbic system. This term was initially coined by the French anatomist Paul Pierre Broca in 1878,3 who used the name the “grand lobe limbique,” which means the lobe on the margin or rim, to refer to cortical regions on the medial edge or rim of the cortical mantle; over subsequent decades it became clear that many of these medial cortical regions are involved in emotional function. The concept of the limbic system has subsequently undergone many revisions since it was first described in detail in the classic paper of James Papez in 1937, and expanded and popularized by Paul MacLean in the 1950s.4 The most restrictive definition of the limbic system includes only the gyrus fornicatus (the cingulate gyrus, retrosplenial cortex, and parahippocampal gyrus), the hippocampus, and the amygdala; based on a range of experimental data and behavioral observations gathered in animals and humans, these regions were initially deemed to represent the neural substrate of emotion. Because of the observation that emotional responses are tightly linked with endocrine and autonomic functions that are largely controlled at the central nervous system level by the hypothalamus, additional regions that have significant connections with the hypothalamus (including the anterior thalamus, septum, and substantia innominata) were subsequently included in the limbic system concept. Later the limbic system was expanded further to include telencephalic and midbrain targets of the descending fibers of the medial forebrain bundle, the “limbic forebrain-midbrain circuit,” with the addition of the habenular complex, the ventral tegmental area (VTA), and dorsal raphe nuclei, among other areas.5 Also, because the ventral striatum receives projections from the amygdala, VTA, and anterior cingulate gyrus, the ventral striatum has been referred to as the “limbic striatum.” In general, a region has been called “limbic” if it appears to be a part of the circuitry involved in the comprehension of the emotional meaning of information in the environment or in the generation of emotional and related autonomic responses. However, it has been argued that many of the regions included in this designation are not primarily involved in emotion generation (e.g., the hippocampus participates in episodic memory processes and spatial navigation, but not directly in emotional responses).6 Also, distinct net-works of regions may be involved in the production of different types of emotions, such as fear, joy, or sadness. However, the general concept of the limbic system has been largely retained by the majority of neuroscientists for convenience, in order to indicate brain regions that process emotional information and are thus involved in the plan-ning, selection, and implementation of survival-promoting behaviors.
PREFRONTAL CORTEX
The portion of cerebral hemisphere anterior to the central sulcus is termed the frontal lobe (Figure 71-1). The frontal cortex is expanded significantly in primates, especially in humans. For purposes of definition, anatomists distinguish the primary motor cortex on the precentral gyrus from the rest of frontal cortex, which is termed prefrontal cortex (PFC) (see Figure 71-1). The nomenclature proposed by the German neuroanatomist Korbinius Brodmann is still in use, and the primary motor cortex is commonly called Brodmann’s area 4 (BA4). Several PFC areas are also part of the motor system that controls striated muscle activity in the body: premotor cortex (BA6), supplementary motor area (medial BA6), presupplementary motor area (medial BA8) and frontal eye fields (BA8) on the dorsolateral surface of the frontal lobe, and cingulate motor areas on the medial surface.
The remaining PFC areas constitute a large expanse of association cortex, which defied classification and elucidation until the era of modern neuroscience. The term association cortex simply refers to those cortical areas that are not directly responsible either for sensory processing or for motor planning. In fact, it was recognized more than a century ago that damage to the PFC does not cause any focal abnormalities in sensory or motor function; rather, PFC lesions cause diffuse cognitive deficits and personality changes. Starting in the 1950s, advances in anatomy, physiology, and behavioral science showed that the PFC is not an amorphous association cortex that supports diffuse “higher functions”; instead, its orderly connections with other brain regions allow the PFC to subserve functions as diverse as spatial working memory, emotional vocalizations, and taste perception. In order to understand this diversity, it is best to consider three sectors within the PFC separately: the orbital PFC (often called the orbitofrontal cortex, or OFC), so called because of its location directly superior to the orbits of the eyes; the dorsolateral PFC (DLPFC); and the medial PFC (mPFC) (which for our purposes includes the anterior cingulate cortex) (see Figure 71-1).
We will review each of these sectors in turn, while keeping in mind certain overall themes: the PFC receives significant innervation from monoaminergic systems, including dopamine and serotonin; each sector of the PFC has reciprocal relationships with a specific portion of the mediodorsal thalamic nucleus; and, like all cortical areas, PFC areas send a massive unidirectional projection to the striatum, which then funnels inputs to the pallidum and from there to the thalamus (Figure 71-2).
Orbitofrontal Cortex
The OFC is the cortex on the ventral surface of the frontal lobe. It is bounded by the insula and the basal forebrain posteriorly, the frontal pole of the brain anteriorly, the gyrus rectus medially, and the lateral frontal gyrus laterally (see Figure 71-1). There is a gradient of cortical differentiation within the OFC, with more posterior areas being more primitive agranular (i.e., lacking layer 4), five-layered cortex, while more anterior areas being well-differentiated six-layered cortex. This contrast is also reflected in the connections of the OFC, since sensory inputs from all five modalities and the viscera arrive at the posterior orbital areas, while direct sensory inputs into the anterior OFC are few. Olfactory and gustatory information reaches the posterior OFC via the primary olfactory and gustatory cortices located just posterior to the OFC, mostly within the insula. More highly processed visual, somatosensory, and auditory information arrives in the OFC from parietal and temporal unimodal association areas devoted to each sensory modality. The somatosensory information arises from somatosensory cortex devoted to the mouth and hands, indicating a possible relationship to feeding. Finally, viscero-sensory information (denoting fullness) is relayed to the OFC from the nucleus of the solitary tract, via the ventroposterior medial thalamus and agranular insula.
Sensory inputs from each modality arrive at adjacent, but architectonically distinct, cortical areas within the posterior OFC. Each of these cortical areas then projects to more anterior OFC areas that integrate inputs from multiple modalities. Thus, there is a hierarchy of processing in the OFC, with anterior OFC areas receiving multimodal sensory information, most of which appears to be related to appetitive or to reward-related behaviors (e.g., food and feeding-related behaviors in rats and nonhuman primates).7
No strong projections from the OFC to motor areas exist. Instead, projections from OFC areas fan out to the medial PFC (see below), and a range of subcortical targets (including the thalamus, striatum, MTL, hypothalamus, and brainstem). The entire PFC is reciprocally connected with the mediodorsal nucleus of the thalamus (MD), but each PFC sector appears to connect with a specific segment of the MD. The OFC receives strong projections from the central portions of the MD, and projects to the same region, creating the possibility of reverberating activity loops in this circuit (see Figure 71-2). In addition, the OFC projects to a central band in the rostral striatum, including the ventrolateral head of the caudate nucleus, dorsal nucleus accumbens, and medial putamen. This band is distinct from the striatal targets of the other PFC sectors, and projects to the ventral pallidum, which in turn innervates the MD. Thus, the OFC is embedded in a cortico-striato-pallido-thalamic loop similar to that described for the motor system.8 The OFC also has largely reciprocal connections with the basal, accessory basal, and lateral nuclei of the amygdala, the subiculum, the entorhinal and perirhinal cortex, and the cortex of the temporal pole. Finally, the OFC sends a projection to the hypothalamus, as well as the periaqueductal gray (PAG) and other midbrain and brainstem areas, but this projection is significantly weaker than that from the mPFC (see below). This projection also innervates the VTA and dorsal raphe nuclei, where dopaminergic and serotonergic neurons are located, respectively.
Studies in both rats and monkeys show that cells in the OFC respond not only to the sensory properties of stimuli, but also to their affective significance, value, or priority. For example, the firing of neurons in the rat orbital cortex during an associative learning task codes better for the reward properties of a smell than for the identity of the smells.9 In experiments on monkeys, neurons that fire strongly at the sight of a desirable food item while the animal is hungry have much lower response levels when the animal is satiated for the same food item. Many of these neurons are still responsive if other appetizing food items are presented. Although the OFC is part of a wider “reward” circuit (including limbic structures, the ventral striatum, and midbrain dopamine neurons), the OFC is unique among these areas in coding for both the identity of sensory stimuli and their rewarding properties.10
Thus, the OFC is a cortical area that is ideally located to receive and to synthesize sensory information related to the rewarding properties of stimuli. In animals, food and feeding-related information is prominently represented in the OFC; in humans this is likely to extend to other kinds of rewarding stimuli. However, the OFC lacks the connections to prepare a motor program in response to the salient features of sensory stimuli it detects. Rather, it conveys this information to the mPFC, and to multiple brain areas involved in generating programs for action, including the striatum and hypothalamus (where fight-or-flight responses can be evoked), as well as to cell groups responsible for the release of dopamine and serotonin. In human neuroimaging studies, the OFC is activated during sensory processing of olfactory and gustatory stimuli, as well as during the determination of pleasantness and aversiveness of these stimuli.11 Thus, it appears that the OFC is involved in the processing of appetitive and aversive aspects of sensation in humans. Also, changes in activity of this brain region have been repeatedly implicated in the pathogenesis of anxiety and mood disorders. For instance, functional neuroimaging studies of subjects with OCD have found abnormally increased OFC metabolism at rest, as well as OFC activation during symptom provocation.12 In addition, subjects with bipolar disorder exhibit abnormalities in the activation of the OFC.13 These findings indicate that the OFC likely plays a major role in the regulation of emotional activity in the brain.
Medial Prefrontal Cortex
The mPFC covers the entire medial surface of the frontal lobe, wrapping around the corpus callosum ventrally, anteriorly, and dorsally (see Figure 71-1). Similar to the OFC, there is a gradient of cortical differentiation within the mPFC where the ventroposterior mPFC areas and the anterior cingulate cortex (ACC) adjacent to the corpus callosum consist of five-layered cortex, while the more anterior areas close to the frontal pole are six-layered cortex. Here we include the ACC as part of the mPFC because of its anatomical location; however, many authors consider it phylogenetically and functionally distinct. The ACC is located on the cingulate gyrus, which wraps around the genu of the corpus callosum dorsally (the dorsal ACC is more closely related to attention and cognition) and ventrally (the subgenual ACC is implicated in mood disorders and emotional regulation).
Inputs to the mPFC arise mainly from other cortical areas: ventral mPFC areas (BA25) receive inputs from the OFC, dorsal mPFC areas (BA9) from the DLPFC, and the ACC (BA24) from other mPFC areas, as well as from the posterior cingulate cortex and parietal and temporal association cortices. The mPFC also receives relatively strong innervation from dopaminergic and serotonergic fibers. Outputs from the mPFC include projections to the medial portions of the MD thalamus, the basal nucleus of the amygdala, and lighter projections to the entorhinal cortex and subiculum. Within the mPFC, BA25 on the ventral medial wall provides the only substantial projection from all of the cerebral cortex to the shell of the nucleus accumbens.14 BA25 and other mPFC areas also project to a ventromedial zone in the striatum (including the medial caudate nucleus, the core of the nucleus accumbens, and the ventral putamen).
There is a unique relationship between the mPFC and subcortical brain areas related to the autonomic nervous system. In primates, the mPFC provides the major cortical input to the hypothalamus, the PAG, and the dopaminergic, serotonergic, and noradrenergic brainstem nuclei. The mPFC projections to the hypothalamus and PAG are organized topographically. For example, the ventral mPFC (particularly BA25) projects to the ventromedial nucleus of the hypothalamus and the dorsolateral PAG, while the ACC projects to the dorsal hypothalamus and the lateral PAG. This is important because different regions within the hypothalamus and the PAG are associated with distinct functions (such as aggression, feeding, sexual behavior, thermoregulation, and anti-nociception). Such functions may occur separately, but they are often evoked together as parts of coordinated coping strategies. For example, stimulation of the lateral column of the PAG by excitatory amino acids generates tachycardia, hypertension, and analgesia as part of an overall confrontational behavioral stance.15 Specific regions within the mPFC can therefore evoke a coordinated array of responses, and these responses will be distinct based on the part of the mPFC that is activated. This relationship between the ventral mPFC and subcortical autonomic areas has led some to term the ventral mPFC “visceromotor cortex” since it exerts an influence on the cardiovascular, gastric, and respiratory systems.16
Its anatomical connections indicate that the mPFC is not a sensory-processing region, as is the OFC. Rather, the mPFC receives OFC and other inputs that highlight the emotionally salient aspects of sensory stimuli, and generates a range of coordinated responses to them. Depending on the challenge, the response can include behavioral and autonomic arousal consistent with a confrontational stance, or behavioral and autonomic slowing for a quiescent stance. The abolition of emotional vocalizations during maternal separation by ACC lesions in monkeys provides an example of the role played by the mPFC in generating emotional reactions to stimuli.7
The ventral mPFC has other interesting properties: human neuroimaging studies show that this region is active during resting conditions, but it becomes deactivated when the individual engages in a variety of cognitive tasks.17 This is interpreted as evidence that, in a resting state, the mPFC is scanning the internal milieu for salient information and this function is taken “off-line” temporarily during a cognitive task requiring attention to the external environment. While the ventral mPFC is primarily involved in autonomic, emotional, and behavioral functions, dorsal parts of the mPFC, especially the dorsal ACC, have been implicated in a variety of cognitive operations, such as attention and error-monitoring processes.18
The mPFC is clearly involved in many brain functions of interest to psychiatrists. Indeed, multiple abnormalities in the mPFC are reported in mood disorders, schizophrenia, substance use disorders, and other psychiatric conditions. For example, the region of the mPFC that is ventral to the genu of the corpus callosum is implicated in the pathogenesis of major depressive disorder and bipolar disorder. Subjects with these disorders show significantly reduced volume and metabolism in this brain area, and this abnormality is associated with a reduction in the number of glial cells in postmortem studies.19
Dorsolateral Prefrontal Cortex
The dorsolateral prefrontal cortex (DLPFC) includes the entire cortex lying on the dorsolateral surface of the frontal lobe, bounded anteriorly by the frontal pole, posteriorly by the motor areas previously mentioned, dorsally by the dorsomedial convexity, and ventrally by the ventrolateral convexity (see Figure 71-1). It covers a large expanse in humans, typically on three gyri termed the superior, middle, and inferior frontal gyri. Almost all of the cortical areas within the DLPFC are six-layered, well-differentiated cortex. One region of special interest is the inferior frontal gyrus of the left hemisphere, also known as Broca’s area (BA44, BA45, and some of BA47). This region’s strong connections with superior temporal cortex (Wernicke’s area), and projection to premotor areas (in the context of mediating language functions), are typical of the pattern of connections of the DLPFC as a whole (see below).
The DLPFC receives significant cortical inputs from multimodal association cortices. Dorsal parts of the DLPFC are connected with the parietal lobe, and receive somatosensory and visuospatial inputs (from the so-called “where” visual stream), while ventral parts of the DLPFC are connected with the temporal lobe and receive auditory and visual object-related inputs (from the so-called “what” visual stream). The DLPFC, like the rest of the PFC, also receives strong neuromodulatory inputs from dopaminergic and serotonergic fibers. Outputs from the DLPFC to other cortical areas mainly target nearby motor, premotor, and supplementary motor areas. Like other PFC sectors, the DLPFC is connected with a specific part of the MD thalamus (the lateral aspect) and the striatum (anterior dorsolateral striatum). Its projections to the MTL are weaker than those of the OFC and mPFC, and mainly target the entorhinal cortex and subiculum. The DLPFC does not project to the hypothalamus, the PAG, or the region of the dopaminergic or serotonergic cell bodies.
Given its connections, it is not surprising that the DLPFC is more closely associated with cognitive functions, and less so with emotional and autonomic processing. One very well-understood aspect of DLPFC function is its participation in spatial and object working memory.20 In a series of classic experiments, monkeys were trained to notice the location of a light shown on a screen, to keep this location in mind during a brief delay period (e.g., 45 seconds), and to subsequently point to the correct location on the screen. During this paradigm, three patterns of neuronal activity are seen in the DLPFC: one set of neurons fires when the light is shown; a second set is active only during the delay period; and a third set fires just before the initiation of the move-ment to point to the light’s location. The second set of neurons is especially interesting, as these keep the information “online” (as it is needed to carry out a subsequent task). This information, termed working memory, is not encoded into long-term memory, but it is essential for normal cognitive function. The working memory neurons are found most typically in the DLPFC, but some are also present in the caudate nucleus and the MD thalamus, both recipients of DLPFC projections. In parallel experiments where the identity of an object, and not its location, needs to be remembered, the working memory neurons are found in a more inferior location in the DLPFC.21 This is consistent with the anatomical connections between the “what” visual stream and the inferior DLPFC. Lesions of the DLPFC cause impairment in working memory tasks in nonhuman primates, as well as in humans.
Working memory is crucial not just for holding new information online, but also for pulling up relevant past information from memory stores and processing it in light of current needs. In addition, the ability to hold multiple bits of information online as one compares the risks and benefits of several behavioral choices provides for significant behavioral flexibility. DLPFC lesions also lead to impairment in both of these processes, as well as in “executive functions,” which include making plans into the future and putting multiple actions in order to achieve a specific goal (as in the Tower of Hanoi puzzle). Of greater relevance to psychiatry, even subtle DLPFC dysfunction results in impoverished behavioral repertoires, reduced behavioral flexibility, and perseveration. One unifying feature of the cognitive operations subserved by the DLPFC may be the organization of behavior in the temporal domain (e.g., the ability to plan into the future, to predict the consequences of future actions, and thus to engage in hypothetical-deductive reasoning).22
Abnormalities in the DLPFC are reported in a variety of psychiatric conditions. In attention-deficit/hyperactivity disorder (ADHD), disrupted cognitive and behavioral integration is associated with abnormal activation of the DLPFC. In schizophrenia, the concept of “hypofrontality” was proposed to explain the impoverished and perseverative cognition and working memory deficits of the disorder. Significant neuroimaging and postmortem abnormalities are reported in the DLPFC in schizophrenia, and to some extent in bipolar disorder, which may underlie some of these functional deficits.23
Prefrontal Cortex: Summary and Conclusions
The PFC is a large and complex brain region that is best conceptualized as being located at the highest point of a sensorimotor pyramid, starting with the primary sensory cortices and ending at the primary motor cortex (Figure 71-3). Highly processed information from sensory association areas converges onto the PFC, which then integrates the information with existing priorities, leading to the construction of adaptive behavioral plans based on this input. Different PFC sectors receive different sensory information and project to different effector areas, but the pattern is consistent. The DLPFC contains its own sensorimotor transfer machinery, while the OFC and mPFC are located in the same sensorimotor circuit (with OFC receiving sensory inputs, transferring relevant information to the mPFC, which then generates appropriate reactions).
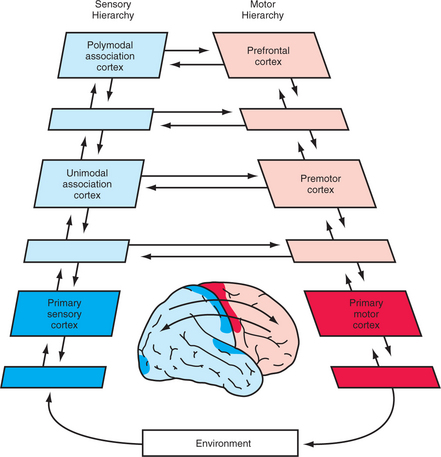
Figure 71-3 The place of the prefrontal cortex within the sensorimotor processing hierarchy of the human brain.
It can be said that linking sensory inputs to motor outputs is a function of the entire brain, but the PFC is uniquely located to guide complex cognitive and emotional behaviors using this process. Visceral sensory inputs are particularly important in this regard since they provide information about the status of the internal milieu. Animals with lesions of the PFC (especially the OFC) experience an “interoceptive agnosia,” an inability to determine how the body reacts when faced with contrasting behavioral options and to decide which action is the most desirable. This agnosia then leads to a variety of interlinked emotional, social, and cognitive deficits.24 For example, loss of the ability to inhibit inappropriate responses, with impaired reinforcement mechanisms in the face of reward contingencies, and impaired reversal learning, may all be explained by this agnosia. These features undoubtedly make it difficult for organisms to survive in complex social networks, as evidenced by the fact that in free-ranging settings primates with PFC lesions lose their position in the social hierarchy, or fall out of the troop altogether and die in solitude.25
The best known example of the consequences of PFC lesions in humans is the nineteenth-century story of Phineas Gage, a respected railroad construction foreman who sustained damage to most of his PFC in a work accident (Figure 71-4). After the accident, Gage became impulsive and socially inappropriate. Despite apparently having sustained no focal neurological deficits and possessing all of his intellectual faculties, he lost his job, strained his family ties, and was left a changed person.26 More recently, the experience with iatrogenic PFC lesions (as a result of frontal leucotomy) showed that humans with these procedures experience a loss of emotional regulation that manifests itself either in apathy toward emotional stimuli or in disinhibition and inappropriate intrusiveness. Although more subtle, many cognitive abnormalities were also reported in these patients, consistent with the likelihood that “cognitive” areas of the PFC were damaged by these lesions.
The magnitude and extended connectivity of the PFC are part of what makes the human brain unique. Since its func tions are so important to human behavior, it is not surprising that multiple psychiatric conditions are associated with abnormalities in the PFC. On the other hand, it is important to note that the PFC is one way-station in a closely coordinated circuit of brain areas. The interactions of the PFC with the striatum, the MD thalamus, and the MTL are essential for proper PFC function. Many consequences of PFC lesions in humans and animals can be replicated by lesions of the striatum, thalamus, or amygdala, or by disconnection of the PFC from these areas.