Fig. 14.1
The therapeutic potential of mesenchymal stem cells (MSC)-secreted factors for treating stroke. Following administration, MSC migrate to the site of stroke in response to chemoattractants, where they release paracrine factors that act to protect and promote repair of the infarcted region as well as surrounding penumbra. The aggregate action of individual paracrine factors that modulate discrete molecular pathways more completely addresses the complex pathophysiology of stroke compared to treatment with any one individual factor
The cumulative evidence supporting a paracrine mechanism of action of MSC does not preclude the involvement of direct cellular contact, which appears to be required in some circumstances, such as immunomodulation (reviewed in [22]). The local concentration of factors also may be enhanced by homing and retention of delivered cells in response to gradients of chemoattractants produced by injured tissues. Furthermore, it has been suggested that, following entrapment, therapeutic cells may respond to local stimuli by modulating the levels of secreted factors in beneficial ways [12, 20, 23, 24]. While certain signaling molecules (for example, tumor necrosis factor-alpha) have been shown to influence stem cell physiology in vitro, there is limited direct evidence for this phenomenon in situ, especially given the transient nature of this effect due to clearance of the bulk of administered cells [25].
It is possible that the potential of pluripotent and multipotent stem cells for replacing tissues will ultimately be realized; however, until that time, present cellular therapies with autologous or allogeneic MSC are providing medical benefits to previously intractable diseases. Understanding the actual potential and limitations of present cellular therapies will be key to gaining widespread adoption.
The Therapeutic Potential for Stroke of MSC-Derived Conditioned Medium
There is increasing interest in developing neurorestorative treatments for acute cerebrovascular injuries, of which acute ischemic stroke (AIS) is the most severe manifestation. Since the cascade of injury in AIS is mostly complete within 24–48 h, neuroprotection, to be most effective, must be started early following the injury, which is challenging in clinical practice [26]. On the other hand, in restorative treatments, the target of the therapy is to promote repair processes such as angiogenesis, neurogenesis, and synaptogenesis. The window for effective promotion of such restorative processes is not precisely known, but may extend to 1 week or 1 month or even longer [27, 28].
AIS involves destruction of multiple cell types including neurons, astrocytes, oligodendrocytes, endothelial cells, and pericytes. Therefore, regenerative strategies will address both neural elements and supportive structures such as blood vessels and glia. Evidence has recently emerged of endogenous repair mechanisms which are activated following cerebral ischemia. Nestin is upregulated in astrocytes after cerebral ischemia [10, 29]. In rodents, subventricular progenitor cells proliferate after middle cerebral artery occlusion (MCAO) and migrate to the striatum where they contribute to the formation of striatal medium-sized spiny neurons and glial cells [10]. However, most of these neuroblasts undergo apoptosis and death. The subventricular progenitor cells continue to migrate to the striatum for at least 4 months after ischemia. This migration is directed by a gradient of stromal-derived factor (SDF)-1 upregulated in the ischemic tissue and CXCR4 expressed on the migrating neuroblasts [4]. SDF-1 is upregulated in astrocytes and endothelial cells for at least 1 month after cerebral ischemia, and also serves to direct the migration of bone marrow (BM)-derived cells involved in tissue repair [1]. Importantly, these endogenous repair mechanisms operating after cerebral ischemia are insufficient for recovery of lost tissues and deteriorated neurological functions. Stimulating this endogenous response with trophic factors is a practical approach directed to augmenting activity of these cellular targets, which are richest immediately (days) following stroke during processes of neural remodeling.
Several cell therapies in early clinical trials build on a large body of preclinical work with MSC isolated from a variety of mesodermal tissues. MSCs improve functional outcome in a dose-dependent fashion in rodent MCAO models when given intracerebrally, intra-arterially, or intravenously [2, 3, 30–35]. Intravenous transplantation effectively improves functional outcome even when given as late as 1 month after the insult [10]. In that instance, there was no reduction in infarct size; instead, there was a neurorestorative effect with increases in angiogenesis, neurogenesis and synaptogenesis in MSC-treated groups [1, 4]. The mechanism of action is not direct cell replacement, but rather the action of MSC as paracrine support factories, elaborating a transient burst of synergistic trophic and growth factors before being cleared [6]. Protective effects observed are anti-apoptosis of injured neural cells, reduced sensitivity to glutamate, suppressed levels of inflammatory molecules, and increased migration and survival of neuroprogenitors [8, 9, 33, 36].
Conditioned Medium: A Potential Cell-Free Stem Cell Therapy
Given the potential therapeutic effects of MSC-secreted paracrine factors as well as available experimental data, we and others have explored the therapeutic potential of conditioned medium (CM) from cultured MSC. This cell-free cocktail of trophic and growth factors has the potential to be safe and effective for treating many diseases currently under investigation as targets for MSC therapies. The combination of factors found in CM reproduces the beneficial effects of MSC. As opposed to delayed secretion in the context of cell therapy, the factors in CM are immediately available to modulate pathology, which is especially critical during the acute phases of diseases such as stroke. Repeated administration is also possible without the risk of rejection by the host. Finally, an approved drug based on this cocktail (especially if lyophilized) may be even more practical and cost-effective than cellular therapies for the reasons that maintenance of cell viability during storage and revival as well as the requirement to remove cryoprotectants (i.e., dimethyl sulfoxide, DMSO) before administration will be obviated.
The functional categorization of therapies based on CM lies between cell therapies and single-factor recombinant protein therapies. A variety of single-factor approaches have been proposed to repair and restore the brain after stroke. One initially promising approach tested hematopoietic growth factors, including erythropoietin or granulocyte colony-stimulating factor (G-CSF), to stimulate neurogenesis. However, both erythropoietin and G-CSF failed in clinical trials in acute stroke [36, 37]. These failures are consistent with the inability of individual agents, affecting discrete signaling pathways, to overcome the extensive damage to multiple cell types and pathways occurring in stroke, at least when administered in tolerable doses. For example, basic fibroblast growth factor (bFGF) was promising in initial studies but was not tolerable in clinical trials due to off-target effects of hypotension and renal toxicity [26].
A potential advantage of CM over single proteins is that the cocktail of factors function synergistically by modulating multiple pathways in concert at much lower (i.e., safer) doses of each protein. Toxicities of selected factors have been related to the relatively high levels required to achieve effects in the brain which leads to high systemic exposure. Most trophic and growth factors bind to different receptors to produce dissimilar and often opposing effects. For example, at relatively high concentrations, nerve growth factor (NGF) stimulates apoptosis through binding to its alternative low-affinity receptor p75NTR, which is upregulated following ischemic cerebral injury [38]. Additionally, there is a sharp drop-off in effectiveness above optimal concentrations. The concentrations of individual therapeutic factors in an efficacious dose of adipose-derived stem cell (ASC)-CM range between 100- and 1000-fold lower than typically administered for single protein factors [39–41]. For example, a previous study in a rabbit model of peripheral vascular disease determined the optimal effect on revascularization of ischemic tissues was achieved with recombinant human vascular endothelial growth factor (VEGF) doses between 0.14 and 0.33 mg/kg body weight [41]. This is compared to an effective dose of CM used in a similar model that contained approximately 0.001 mg VEGF/kg body weight [39]. The lower levels of factors in CM provide for an increased safety factor at effective doses by minimizing off-target effects that may be noted at higher doses of single agents.
The exact form of factors comprising CM is not well understood; however, it is likely that the bulk is complexed with proteins or other macromolecules such as lipids and nucleic acids. One such complex could be microvesicles, such as exosomes, released from the cells in response to environmental cues. It was recently shown that exosomes isolated from MSC-CM potently induced functional recovery following experimental stroke in rats [42]. The exosomes acted to increase vascularity, neurogenesis, and neurite remodeling. A significant portion of the neuroplasticity observed could be attributed to a single microribonucleic acid (microRNA) species rather than protein factors [43]. The discovery that regulatory RNA species may be present in CM and that these activities could contribute significantly to the potency of MSC-CM through complementation of protein factors further substantiates the benefits of a cocktail of factors (as opposed to monotherapies) for treating stroke.
Neuroprotective and Neurorestorative Properties of MSC Secreted Factors
MSC-CM contains both neuroprotective and neurorestorative factors, and engages key molecular pathways involved in each of these processes (Table 14.1). These factors include NGF, insulin-like growth factor-1 (IGF-1), VEGF, glial cell-derived neurotrophic factor (GDNF), hepatocyte growth factor (HGF), brain-derived neurotrophic factor (BDNF), bFGF, and SDF-1 or CXCL12, which bind to cognate receptors expressed on many cell types to promote survival and cellular responses involved in protection and repair [1, 39, 40, 44–48]. Selective inactivation of either BDNF or IGF-1 before administration of ASC-CM in a neonatal model of stroke significantly reduced potency [40]. As expected, IGF-1 in this context acts to protect neurons against inducers of apoptosis via a mechanism involving IGF-1-induced activation of Akt [49]. Activation of Akt provides a pro-survival signal after stroke which attenuates glutamate excitotoxicity and upregulates p-cAMP(adenosine 5′-monophosphate) response element-binding protein (CREB)/BDNF leading to increased cell survival, neurogenesis, and synaptogenesis [50–53]. Further protection is afforded by attenuation of excitotoxicity through stimulating Akt and mitogen-activated protein kinase/extracellular signal-regulated kinase (MAPK/ERK) pathways by multiple factors in CM, including BDNF, HGF, and VEGF [39, 40, 49, 54]. Dendrite formation and synaptogenesis in cultured rata hippocampal neurons were enhanced by soluble factors produced by umbilical- and BM-derived MSC. This activity was partially mediated by BDNF in the CM [55].
Table 14.1
Neuroregenerative and neuroprotective factors secreted by MSC
Functional category | Factor | Mechanisms of action |
---|---|---|
Angiogenic/arteriogenic | ||
VEGF | Endothelial cell mitogen and chemoattractant | |
bFGF | Endothelial cell mitogen and chemoattractant promotes remodeling | |
HGF | Mobilizes vascular and parenchyma forming cells | |
GDNF | Cooperates with VEGF to enhance angiogenesis | |
Pro-survival | ||
VEGF | Blocks endothelial cell apoptosis induced by factors such as TGF-b | |
HGF | Blocks apoptosis through activation of Akt | |
IGF-1 | Blocks apoptosis through activation of Akt | |
BDNF | Protects against glutamate excitotoxicity | |
Neurotrophic | ||
NGF | Stimulates neurogenesis | |
GDNF | Protects many different cell types from apoptosis | |
bFGF | Promotes synaptogenesis | |
Anti-inflammatory/Immunomodulators | ||
PGE2 | Blocks stimulation of many different inflammatory cells | |
IDO | Blocks B cell antibody production. Downregulates IFN-γ | |
HGF | Blocks activation/proliferation of B and NK cells | |
TSG-6 | Suppresses NF-kB stimulation | |
IL-1Ra | IL-1 antagonist | |
sTNF-R | Traps TNF-α to prevent stimulation of cells | |
Recruitment/Mobilization | ||
SDF1 | Chemoattractant through CXCR4 receptor expressed on many cells | |
HGF | Pro-migratory (also known as scatter factor) | |
G-CSF | Promotes cell mobilization |
Neuritogenesis in PC12 cells was also enhanced by treatment with ASC-CM, which was due in part to NGF activation of AMP-activated kinase α (AMPK-α) in vitro [56, 57]. AMPK is the central energy sensor switch, and a key anti-inflammatory regulator of lipid metabolizing enzymes [58]. AMPK activation promotes neuritogenesis and axogenesis during metabolic stress and its loss in supporting structures like oligodendrocytes and astrocytes exacerbates the disease [56, 59, 60]. In the vasculature, AMPK-α1 is upstream to Akt and their cross talk promotes VEGF-induced angiogenesis [61].
Demonstrating the Therapeutic Potential of MSC-CM in Experimental Models of Stroke
Perhaps the earliest demonstration of MSC-CM potency in an animal disease model was by Kinnaird and Epstein [62]. The CM from BM-MSC promoted reperfusion of ischemic tissue in a mouse model of peripheral vascular disease. Treated tissues exhibited reduced muscular atrophy, enhanced vascularity, and reduced necrosis. A number of hypoxia-inducible pro-angiogenic and pro-survival factors were present in the CM and neutralization of two (VEGF and bFGF) only partially reduced the ability of the mixture to promote endothelial and smooth muscle cell migration. A related study by Rehman et al. demonstrated ASC expression of hypoxia-inducible trophic factors, including bFGF, VEGF, and HGF, and observed rapid positive effects after administration in a murine hind limb ischemia model [45]. Subsequently, it was determined that knockdown of HGF expression by siRNA severely attenuated ASC-mediated reperfusion in the same model [63]. In an attempt to increase BM-MSC survival and, thus increase the effective cell dose, the laboratory of Victor Dzau generated cells expressing the transgene for Akt [64]. The transgenic cells showed enhanced ability to promote repair of ischemic myocardium; it was determined that this effect was due to enhanced paracrine activity and that similar effects could be demonstrated by provision of the CM alone [13].
The CM from BM-MSC and ASC have been shown to protect and repair brain tissues in rodent models of focal and global ischemic stroke. A global ischemia neonatal rat hypoxia-ischemia encephalopathy (HIE) model was used to test ASC-CM in a neonatal model of hypoxia-ischemia (HI) injury. A single intravenous injection at up to 36 h post injury significantly reduced brain volume loss and promoted functional recovery at 1 week and 3 months [40]. Neutralization of either IGF-1 or BDNF before injection partially reduced this effect. The salutary effects of ASC-CM were related to protection from glutamate excitotoxicity and protection of neurons from ischemic insult. Similarly, a rat model of stroke induced by MCAO was treated with ASC-CM infused directly into the lateral ventricle [5]. Continuous infusion for 8 days beginning at 1 week after surgery reduced infarct volume and enhanced motor control at up to 15 days following MCAO surgery. This activity was attributed to enhanced microvascularity in the infarct zone as well as reduced cell death and inflammation with treatment. Short-term protection (e.g., 24 h) of a single intracerebroventricular injection of concentrated ASC-CM following temporary MCAO with reperfusion in a murine model was effective in reducing infarct volume and edema [7]. In a study that has implications for treatment of stroke victims with an autologous product, Tsai et al. evaluated CM from BM-MSC isolated from either normal healthy rats or animals that had experienced an induced stroke [30]. Both CM exhibited similar effectiveness in enhancing functional recovery when delivered intravenously. The effect was associated with increased neurogenesis and reduced inflammation within the infarct zone.
Toward Development and Testing of Clinical-Grade MSC-CM
The cumulative data from preclinical studies strongly support the therapeutic potential of MSC-CM for treating stroke as well as other diseases. As with any unproven investigational agent, regulatory approval by governing bodies will be required before commencing trials in humans. These agencies include the US Food and Drug Administration (FDA) and the European Medicines Agency (EMA) as well as local Institutional Review Boards (IRB), and Competent Authorities (CA) in the European Union. This section focuses on the regulatory pathway for new drug approval in the USA.
Possible Regulatory Pathways for MSC-CM
In the USA, an investigational new drug application (IND) is required for nearly all new drugs entering clinical trials. The IND comprises three sections: chemistry and manufacturing controls (CMC), clinical study design, and nonclinical studies. The nonclinical studies section mainly concerns safety and toxicity in animals using the clinically intended route of administration and a product very similar, if not identical, to that which will be used in the clinic. This section typically includes a description of efficacy studies in relevant disease models. The CMC section pertains to manufacturing processes and quality control systems for ensuring consistency and the absence of potentially deleterious agents in the final product. Each of the sections of the IND must provide reviewers with a sufficient amount of detail to determine the potential safety of any product before allowing evaluation in humans.
The regulatory route for licensure of an eventual drug based on MSC-CM will likely require a Biological License Application (BLA) as opposed to a New Drug Application (NDA), the latter which generally pertains to drugs of well-defined composition. Within the FDA there are two centers responsible for oversight and approval of new drugs. The Center for Biologics Evaluation and Research (CBER) and the Center for Drug Evaluation and Research (CDER). Jurisdictional oversight of biologics generally falls to CBER; with important exceptions for less complex entities, such as monoclonal antibodies and recombinant proteins. Therefore, the complexity of MSC-CM, whether whole or partially fractionated, likely will place it under the review of CBER.
Establishing Good Manufacturing Process for Producing MSC-CM
The FDA, as well as other entities charged with overseeing aspects of biologic and cell therapies, has issued guidance documents detailing the requirements that must be fulfilled to create a product with appropriate characteristics (i.e., the CMC component of an IND) [65–72]. This section presents an overview of the guidelines for reagents and materials of acceptable quality for good manufacturing practices (GMP) production of MSC-CM to be used in the clinic.
Sources of Donor Tissues and Cells
Of foremost importance to the manufacturing process is the nature and qualification of cell sources. The FDA has constructed careful guidelines for screening donors for human cell and tissue products (HCT/P) to minimize the chance of introducing adventitious agents into the final drug product [67]. Donors must be qualified for minimal risk of transmitting diseases through the use of both a comprehensive health history questionnaire and blood testing. Infectious agents of concern include human immunodeficiency viruses (HIV), hepatitis viruses, human T-lymphotrophic virus (HTLV), cytomegalovirus (CMV), Treponema pallidum (causative agent of syphilis), Trypanosoma cruzi (causative agent of Chagas disease), West Nile virus, and prion proteins. Following qualification of the donor, additional screening is performed periodically during the manufacturing process.
Ancillary Materials Used in Manufacturing MSC-CM
Ancillary materials (AM), also referred to as ancillary reagents, are substances used in manufacturing of the product but not anticipated to be present in the final product. The control and appropriate qualification standards for AM are intended to reduce the chance of introducing substances into the final product which may transmit disease or induce toxicity in recipients. Generally, AM used for manufacturing should be well characterized and have a certificate of analysis (CoA) containing detailed information regarding source materials and quality testing. An important caveat for MSC-CM is that current somatic cell therapy manufacturing guidance documents were contemplated for a cellular product and not for the medium conditioned by cells. Whereas it is possible to reduce the levels of AM in the final product through washing of the cells, some level of reagents used in the manufacture of MSC-CM will remain a part of the final product, regardless of the processes used. For example, cells grown in a nutrient-rich medium containing proteins, such as animal serum or recombinant growth factors, could be washed thoroughly before transferring to basal medium to remove the majority of these proteins. However, there is invariable carry-through of medium components associated with the cells. Partial purification through ultrafiltration or other means may further reduce the quantities of these agents, but the extent will depend on the nature of the substance and the pore size of the ultrafiltration medium. Thus, certain AM used for the manufacture of MSC-CM will become part of the final product.
The US Pharmacopoeia (USP) monograph on AM (USP < 1043 > ) as well as the International Committee for Harmonization (ICH) guidance provide useful guidelines for selecting appropriate materials to be used in cell manufacturing, and these guidelines would appear to pertain to MSC-CM [65, 69]. Examples of components include enzymes used for cell liberation, media components, disposables employed for cell isolation and separation, and culture flasks. The first tier of AM qualification describes the optimal characteristics of reagent possessing the lowest potential risk of having a negative impact on safety and efficacy of the final product (Table 14.2). These reagents are highly qualified and generally are approved for human use. The next level (tier 2) are generally regarded as low-risk reagents and materials produced in full compliance with current good manufacturing practices (cGMPs), as demonstrated by appropriate documentation in a CoA. Further down on the list and possibly unacceptable for incorporation into cell product manufacturing due to unknown risks of introducing undesirable substances are tier 3 (research or diagnostic grade) AM or the highest-risk tier 4 AM that are not well characterized.
Table 14.2
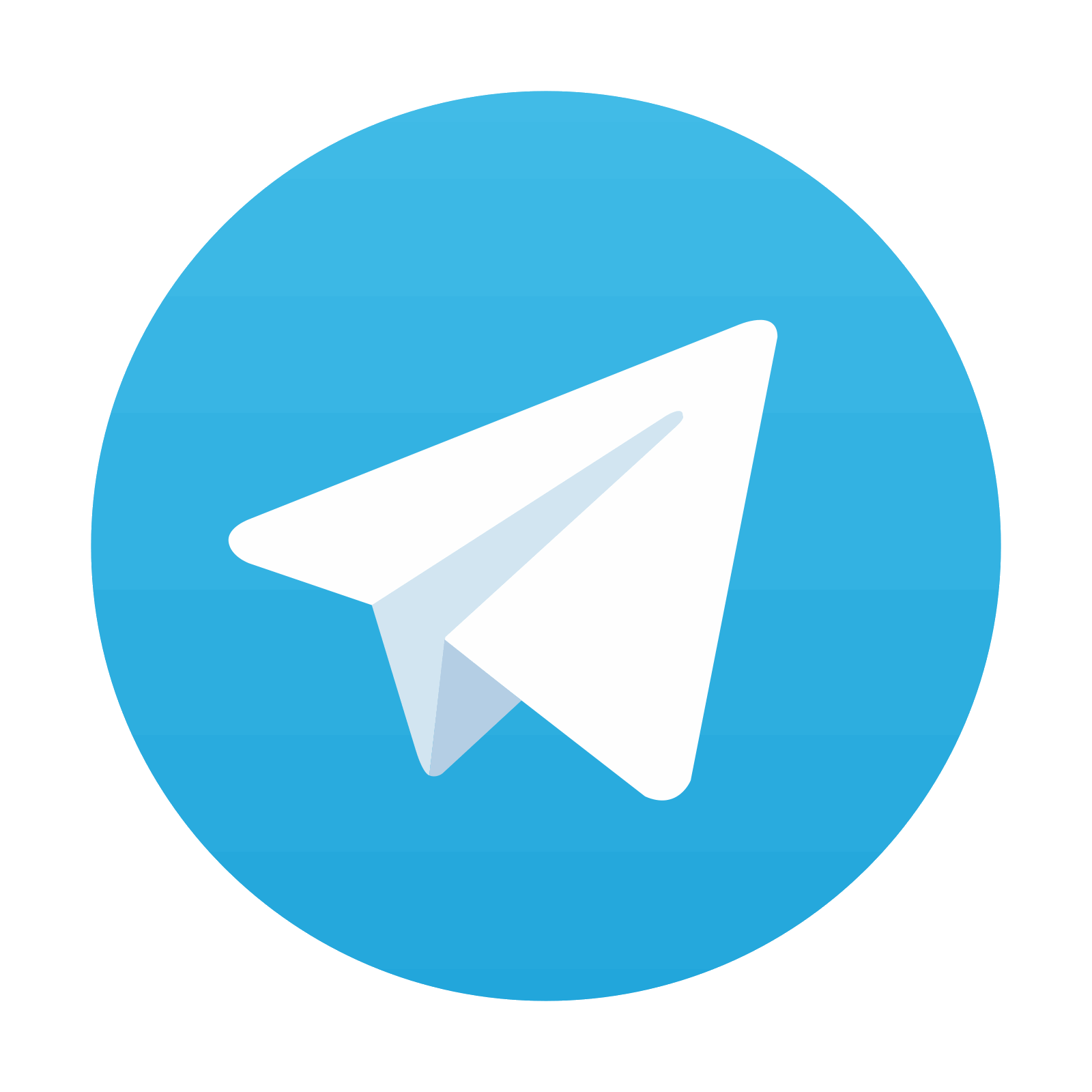
Risk stratification of ancillary materials
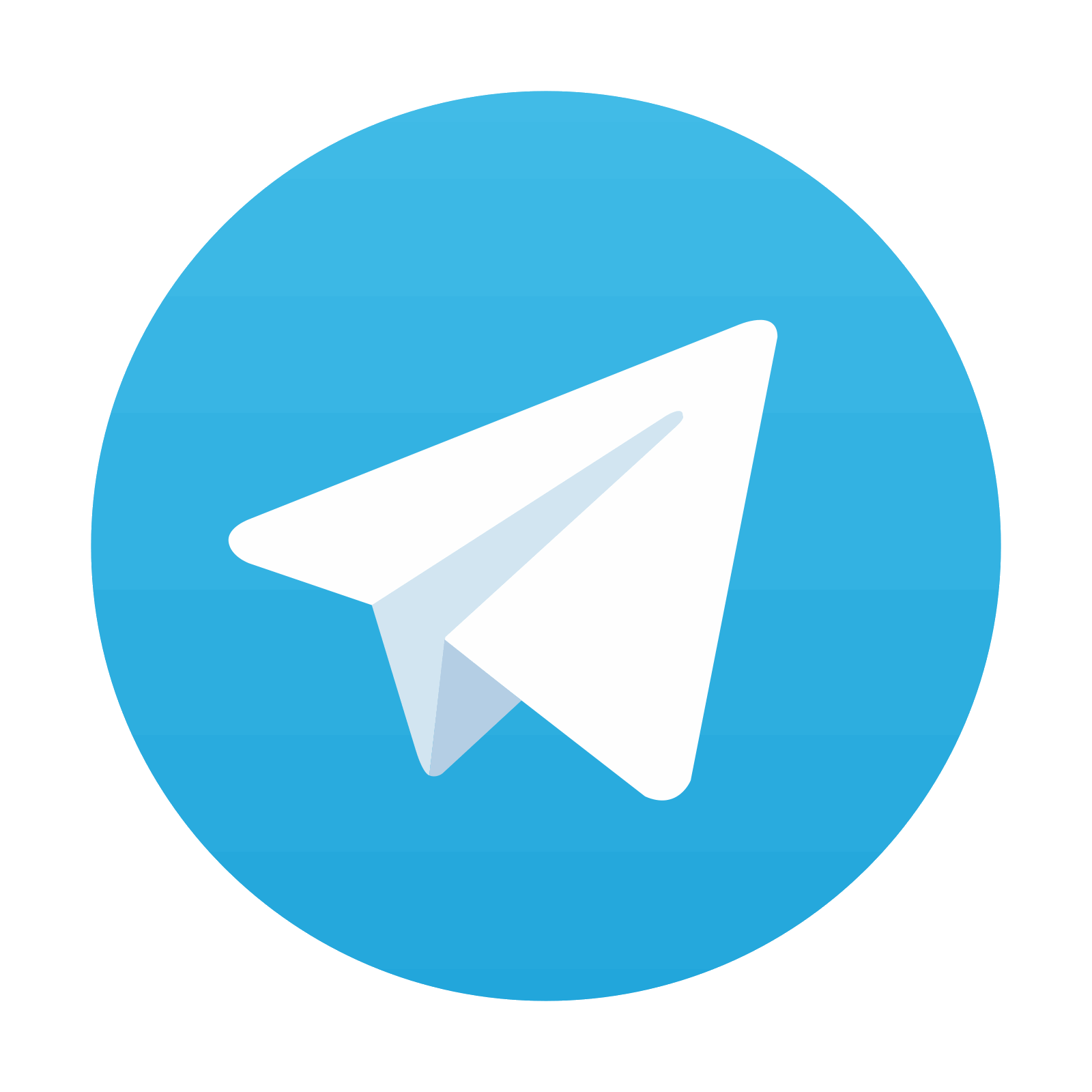
Stay updated, free articles. Join our Telegram channel
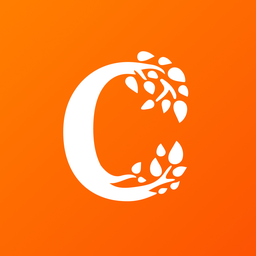
Full access? Get Clinical Tree
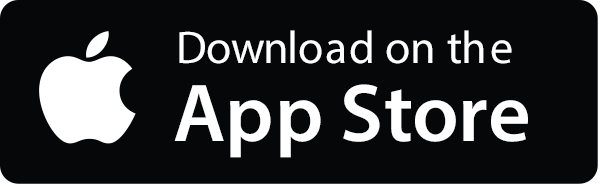
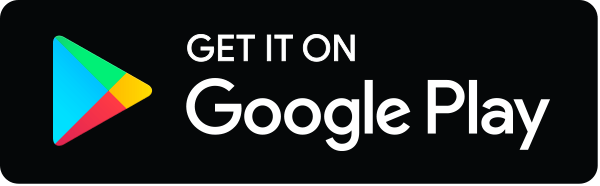
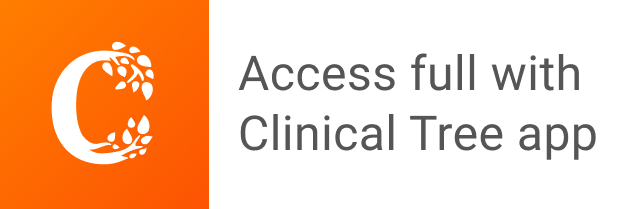