Chapter 40 Animal Models for Disorders of Chronobiology
Cell and Tissue
Abstract
Circadian rhythms are not merely passive reflections of the environmental light–dark cycle, but rather depend upon an underlying internal endogenous clock. Our internal molecular clocks are reset or entrained on a daily basis by zeitgebers (environmental cues such as light or food) that provide information about the external time and allow us to stay in synch with the environment (Fig. 40-1). This allows organisms to anticipate, rather than simply react to, daily changes in the external environment and to synchronize behavioral and physiologic processes to optimize energy use, reproduction, and survival. For example, organisms are primed to optimize anabolic events such as gluconeogenesis in liver during the fasting/rest period to maintain glucose homeostasis in the organism and avert hypoglycemia during sleep. Studies have also demonstrated that chronic reversal of the external light–dark cycles leads to a significant reduction in survival time of cardiomyopathic hampsters,1 further emphasizing the importance of the internal circadian clock in physiology and general health.
Clock Regulation of Physiology and Sleep
It has long been recognized that many aspects of human physiology vary depending upon the time of day. For example, heart attacks, strokes, pulmonary edema, and hypertensive crises all peak at certain times during the day.2,3 Given the invention of artificial light, the advancements and ease of jet travel, and the rampant increase in Internet and television use, people routinely extend their awake time into the night and become desynchronized from their environmental (light) cues. Indeed, in parallel with the significant decrease in sleep time in the United States over the past several decades has been a concurrent increase in prevalence of obesity.4 Numerous cross-sectional and prospective clinical studies have further revealed that reduced sleep duration and quality predicts the development of obesity and type 2 diabetes after various confounding factors were taken into account.5 Finally, diseases related to sleep, such as sleep apnea syndrome, are common in persons with the metabolic syndrome, and treating the sleep apnea has been found to improve energy balance and metabolism.5
Association studies have revealed that shift and night workers also have an elevated risk for the development of obesity and metabolic syndrome.6,7 Repeated jet lag exposure in experimental model organisms results in accelerated cardiomyopathy and premature mortality.1,8 Clinical studies have also revealed that even short-duration sleep restriction (4 hours of sleep for 6 consecutive nights) in otherwise healthy subjects results in impaired glucose tolerance and reduced insulin responsiveness.9 Humans with night-eating syndrome may also be further predisposed to the metabolic syndrome.10 In addition to these examples of chronic misalignment between the cycles of sleep/fasting and wake/feeding, genetic polymorphisms within Clock and Bmal1 have also been shown to be associated with metabolic phenotypes, including obesity, hypertension, and type 2 diabetes.11–13 Collectively, these data suggest that both the time of day and the amount and time of sleep–wake cycles are critical to maintain metabolic homeostasis and the overall health of the organism.
Many metabolic processes display rhythmicity across the light–dark cycle. In particular, circadian control of glucose metabolism has been well documented.14 For example, oral glucose tolerance is impaired in the evening compared to morning hours, an effect that is believed to be due to a combination of both decreased insulin secretion and altered insulin sensitivity in the evening.15 The dawn phenomenon is also a well-described phenomenon whereby glucose levels peak before the onset of the active period.16,17 Destruction of the master circadian pacemaker within the hypothalamic suprachiasmatic nucleus (SCN) abolishes the diurnal variation in glucose metabolism in rats.18 Disruption of the normal cycles of glucose tolerance and insulin secretion is a hallmark of type 2 diabetes patients,19,20 and diabetic rats display loss of daily rhythmicity of corticosterone secretion and locomotor activity.21,22 Persons with type 1 diabetes mellitus are required to adjust their daily insulin injections depending on the time of day, as insulin sensitivity and glucose tolerance fluctuate throughout the day.20 Whether circadian control of glucose metabolism can be attributable entirely to neural centers or instead may involve peripheral tissue clock function is still unknown. Thus, while it is well established that the circadian clock plays a critical role in maintaining normal rhythmicity of physiologic processes, much less understood are the molecular mechanisms underlying these phenomena.
The Core Molecular Clock Network
The first mammalian circadian gene to be identified was Clock, or circadian locomotor output cycles kaput, discovered in a large-scale unbiased chemical mutagenesis screen for circadian variants in mice in the 1990s.23–25 Since then, rapid advances in the field have identified that the core molecular clock network is composed of an autoregulatory transcription and translation feedback loop that generates 24-hour rhythms of gene expression (Fig. 40-2).26 The positive limb of the clock consists of CLOCK and BMAL1 (brain and muscle ARNT-like), members of the basic helix-loop-helix period-ARNT-single-minded (bHLH-PAS) transcription factor family. CLOCK and BMAL1 heterodimerize and activate transcription of downstream target genes, including the period (Per1, Per2, and Per3) and cryptochrome (Cry1 and Cry2) genes, which contain E-box enhancer elements in their promoters. Following translation and posttranslational modifications, the PER and CRY proteins heterodimerize and translocate back to the nucleus, where they inhibit the transcriptional activity of CLOCK/BMAL1, thereby constituting the negative limb of the clock. This entire cycle takes approximately 24 hours to complete before cycling anew. Additional regulatory loops are further superimposed upon this core loop to provide flexibility and adaptability in regulating the circadian clock.27,28 For example, CLOCK/BMAL1 also activates the transcription of the retinoic acid–related orphan nuclear receptors Rev-erbα and Rorα, which inhibit and activate, respectively, transcription of Bmal1 itself.29
Genetic mouse models have revealed key roles for each of the core clock genes in the generation and maintenance of circadian rhythmicity. Mice with a dominant-negative mutation in the Clock gene have an initial lengthening of their free-running period before becoming arrhythmic in constant darkness,24 whereas Bmal1 knockout mice display a complete loss of rhythmicity in constant darkness.30 Per1/Per2 and Cry1/Cry2 double-knockout mice also display arrhythmicity that is much more pronounced than in the single mutants, demonstrating compensation within the negative limb of the clock.31–33
Identification of the molecular components of the core clock complex was key to further understanding how the clock network controls both behavior and physiology in the whole organism. It was initially believed that the master pacemaker in the SCN was the sole clock-regulating rhythms throughout the body. However, pioneering work led by Schibler and others demonstrated that the molecular clock mechanism operates in a cell autonomous fashion in nonneuronal mammalian cells and in most, if not all, peripheral tissues and extra-SCN regions of the brain in addition to the SCN.34–36 The master pacemaker clock in the SCN, which receives light input from the eyes via the retinohypothalamic tract, synchronizes the peripheral clocks, whose phase is approximately 4 hours delayed compared to the SCN (Fig. 40-3, Video 40-1).
Molecular Gene Expression Patterns Governed by the Clock
The core circadian clock components are well defined, but the targets that in turn regulate the rhythmicity of downstream metabolic processes are much less clear. One example of a known clock target is D-element binding protein (Dbp), a transcription factor that binds to the insulin-response element of key metabolic genes involved in gluconeogenesis and lipogenesis.37 Because oscillation of CLOCK/BMAL1 activity in liver induces 100-fold changes in expression of Dbp, it is easy to imagine how this would translate to rhythmic regulation of metabolic processes such as gluconeogenesis.
Microarray analyses have further revealed a plethora of information regarding the vast number of genes that are under control of the clock in the brain and within peripheral tissues. An estimated 3% to 20% of the entire transcriptome displays 24-hour oscillation of gene expression within liver, heart, fat, and SCN, and many of these genes are key rate-limiting enzymes and transcription factors involved in metabolic processes including lipid metabolism, gluconeogenesis, and oxidative phosphorylation.38,39
There is very little overlap of cycling gene transcripts among the different tissues—only 37 genes showed similar patterns of gene expression between heart and liver, suggesting that tissue-specific rhythmic gene expression allows each cell or tissue to function optimally at appropriate times in the light–dark cycle. For example, during the fasting/sleep period, expression of genes involved in glucose production, glycogen breakdown, and fatty acid oxidation are elevated in order to help the organism maintain glucose homeostasis in the fasted state. During the feeding/wake period, there is a peak in expression of the appropriate gastrointestinal tract enzymes that are required for optimal absorption and digestion of nutrients.40 Thus, the circadian patterns of metabolic gene expression may facilitate the switch between the daily cycles of fasting and feeding.
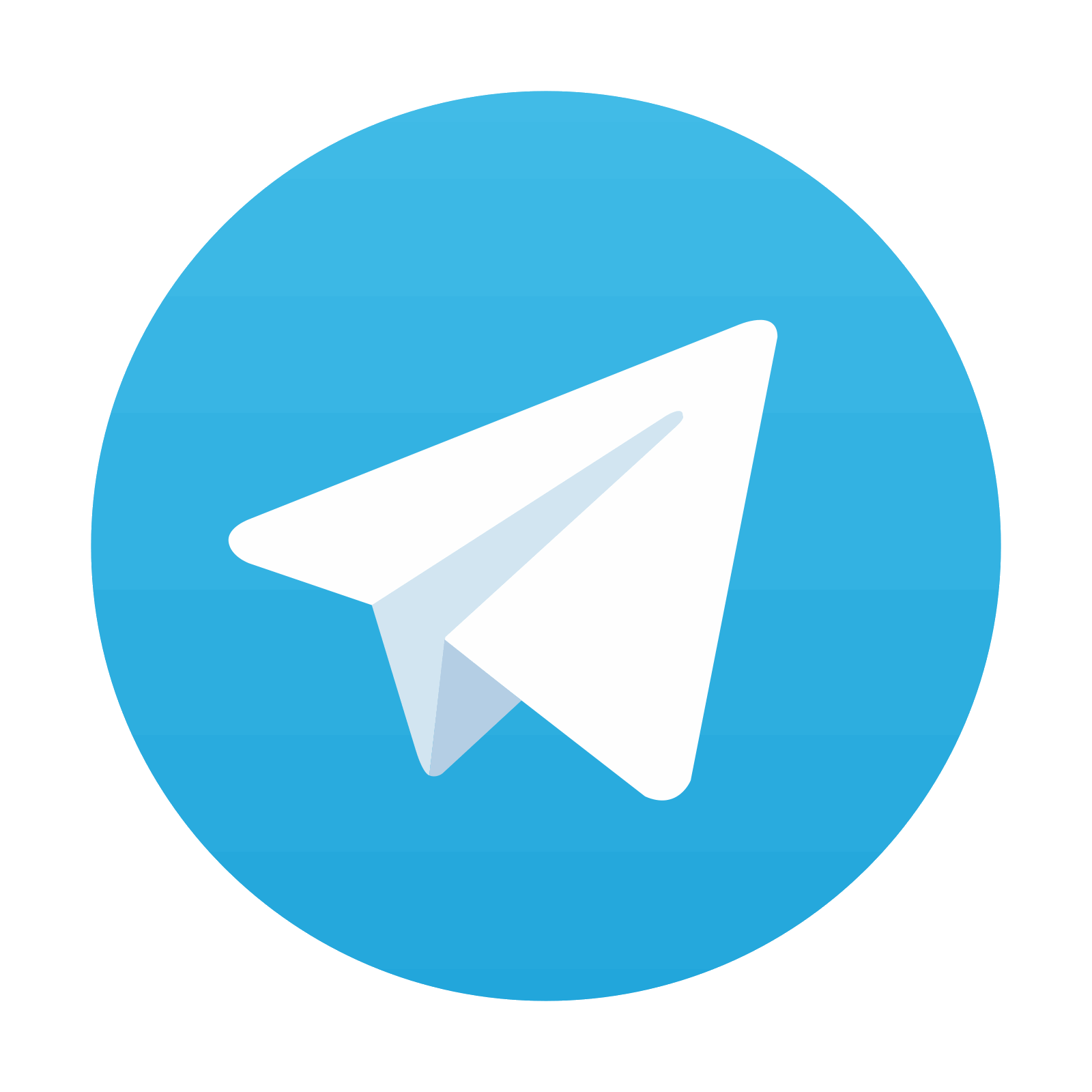
Stay updated, free articles. Join our Telegram channel
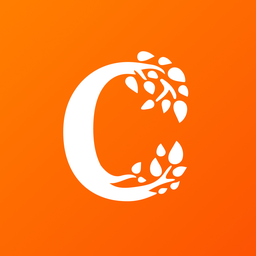
Full access? Get Clinical Tree
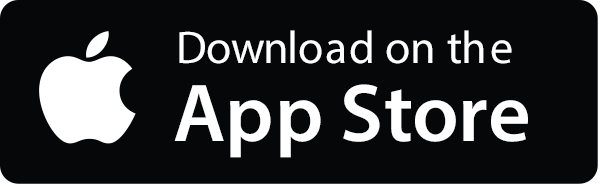
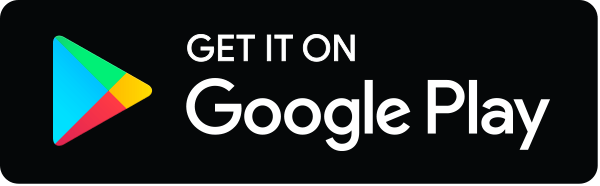