Antiepileptic Drug Development and Experimental Models
Jacqueline A. French
In 1962, the Kefauver-Harris amendments to the federal Food, Drug, and Cosmetic Act ushered in the modern age of drug testing by requiring that pharmaceuticals be proved effective prior to marketing (1). At the same time, federal agencies were given the authority to determine whether the safety and efficacy of a drug had been satisfactorily demonstrated. Although this law prevented ineffective compounds from entering the market, it also significantly impeded the development of new, effective treatments. Antiepileptic drugs (AEDs) were particularly affected because of the difficulties and cost of their trials. After valproic acid was approved in 1978, no new agents were developed for a decade. Meanwhile, 50% of patients with epilepsy had either unacceptable seizure control or side effects that affected their quality of life.
From this clear need for new agents came the National Institutes of Health (NIH) Antiepileptic Drug Development (ADD) Program (2), which screens thousands of compounds against animal models of epilepsy. As of 2002, 24,000 new chemical entities have been screened (S. White, Epilepsy Research Branch NIH, personal communication 2002). A subset of these compounds is further evaluated, and in conjunction with pharmaceutical companies, the most promising agents are tested in humans via clinical trials. Mainly as a result of the efforts of the ADD Program, the 1990s saw many new AEDs approved, with even more agents entered into clinical trials. More recently, many smaller companies have entered the AED development arena. This has placed a greater emphasis on early determination of drug efficacy in order to assess potential return on investments. There is also a greater emphasis on obtaining a monotherapy indication, which is seen as advantageous in the marketplace. These corporate needs, however, must always be balanced against patient safety issues.
This chapter reviews the process by which a compound is discovered, undergoes preclinical and clinical testing, and is ultimately approved for use, with emphasis on AED development in the United States, as directed by the Food and Drug Administration (FDA).
PRECLINICAL DEVELOPMENT
Drug Discovery
Since Merritt and Putnam discovered phenytoin in 1938, identification of AEDs has depended on animal screening of anticonvulsant activity. Under the ADD Program, approximately 1000 compounds from various sources are evaluated each year for potential antiepileptic action. Sometimes pharmaceutical companies send large numbers of random compounds for screening. For example, felbamate was initially synthesized as an analogue of the tranquilizer meprobamate (3,4). Similar screening may be performed within pharmaceutical companies, often on compounds specifically designed as potential anticonvulsants. An example is vigabatrin, an irreversible inhibitor of γ-aminobutyric acid (GABA) transaminase (5). Regardless of its source, however, if a compound demonstrates no effect in animal screening, it will most likely receive no further evaluation, raising concern that agents that act via unusual mechanisms may be inappropriately rejected.
Primary Screening Models
There are two basic strategies for discovering new chemical entities that may be useful for the treatment of epilepsy. The first method is called model-based screening. In this approach, high volumes of compounds undergo chemical screens that are predictive of antiepileptic effect. Compounds that prove effective in these screens will undergo further testing to assess toxicity and to try to elucidate the mechanism of action. Topiramate and felbamate are examples of recent AEDs that were discovered in this manner. Not surprisingly, these agents are frequently determined to have multiple mechanisms of action. The second strategy used for drug discovery is known as mechanism-based screening. Here an attempt is made to synthesize a compound that will have a desired pharmacologic effect, such as GABA enhancement or N-methyl-D-aspartate (NMDA) blockade. Examples of agents recently discovered using this technique include vigabatrin and tiagabine, both of which are GABA enhancers. In most cases, compounds that are discovered based on mechanism of action will still be evaluated using standard chemical screens.
The two primary animal screens used to assess anticonvulsant activity are maximal electroshock (MES) and subcutaneous pentylenetetrazol (scMET). In the MES test, rodents receive 60-Hz alternating current for 0.2 seconds through corneal electrodes and are then observed for tonic hind-limb extension. A preadministered compound that can abolish this response is presumed to have the ability to abolish seizure propagation. In the second screen, pentylenetetrazol is administered subcutaneously. Untreated animals experience clonic spasms within 30 minutes. Compounds that increase seizure threshold can prevent this response (6). These two tests are performed at various intervals after an agent has been administered in order to assess duration of activity. If no anticonvulsant activity is evident on either test, the threshold tonic extension test—which, unlike the MES, uses submaximal rather than supramaximal electroshock stimulation—is performed before the agent is abandoned (6).
It is believed that the MES and scMET tests may, in some way, distinguish the potential utility of compounds against different seizure types. Compounds effective in the MES screen may be more useful in patients with partial and generalized tonic-clonic seizures, whereas those effective in the scMET screen may have activity against absence seizures. Unfortunately, many AEDs prove to be an exception to the rule. For example, gabapentin prevents clonic spasms after pentylenetetrazol administration but has no activity against absence seizures (7,8).
The emphasis on a small number of chemical screening models to discover new agents has been criticized by some individuals. It is possible that this method may uncover only those drugs with similar mechanisms of action and may discard novel drugs with unique properties. Although most new AEDs are effective in either MES or scMET tests, one useful compound, levetiracetam (see Chapter 63) was ineffective in either of these screens. Greater emphasis is now being placed on the use of biologic screens, including kindling models; spontaneous seizure models, such as the genetic absence epilepsy rat from Strasbourg (9) and the audiogenic seizure-prone rat, both of which model genetic spike-wave epilepsy in humans; and models of spontaneous seizures following status epilepticus, such as the perforant-path and pilocarpine-induced status models (10,11). A recent NIH workshop was held to foster identification of new models that might better predict efficacy in the human epilepsy condition (12).
Information from the three tests described is combined with results of neurotoxicity assays, including the Rotorod, positional sense, gait and stance, and muscle tone tests (6), to yield a therapeutic index (TI). The TI is the ratio of the median toxic dose (TD50) to the median effective dose (ED50). A high index is indicative of good tolerability at doses required to control seizures. Again, there has been a new perspective on the older model meant to elucidate toxicity. Toxicity testing is performed using acute seizure model such as MES and scMET. Recently, it was discovered that animals who have undergone kindling may be more susceptible to certain types of side effects than are normal animals. This raises the question of whether patients with epilepsy might also be prone to such side effects. In the future, it is likely that more toxicity testing will be performed in animal models of epilepsy (13,14).
Differentiation of Mechanism of Action
Tests to elucidate possible mechanisms of action include injections of the chemoconvulsants bicuculline (a GABA antagonist), picrotoxin (a chloride-channel blocker), and strychnine (a glycine antagonist). The compound can also be used to determine whether kindling can be prevented in corneally kindled rodents (indicating antiepileptogenesis) or whether seizures can be prevented in fully kindled animals (indicating antiepileptic activity). The results from these tests are becoming more critical, as investigators begin to search for agents that may prevent seizures. It has been suggested that drugs that prevent the acquisition of kindled seizures may have special properties that would prevent the development of an epileptic focus after a brain injury, such as stroke or head trauma. To date, however, such effects have not been proven. Other useful screens include the Frings audiogenic seizure-susceptible mouse, and spike-and-wave discharges provoked by γ-hydroxybutyrate (15), a possible screen for agents that have anti-absence potential (6). Genetic mouse models may also be useful for this purpose. Finally, in vitro tests can search for specific receptor binding and for the ability to block sodium, chloride, or calcium channels (16).
Tolerance and Pharmacokinetic Properties
Early in the evaluation of a compound some pharmacokinetic information can be obtained. Chronic dosing studies coupled with anticonvulsant screening tests can provide information on whether tolerance may develop. Liver enzyme tests can indicate toxicity and effect on hepatic metabolism, including inhibition of the cytochrome P450 enzyme. Liver tests may also help to establish the route of metabolism (e.g., oxidation or glucuronidation) (6).
Toxicity
Toxicity testing of AEDs is regulated by the FDA. Before single-dose studies in humans can be initiated, acute toxicology studies must be conducted using three dose levels in at least two animal species for a minimum of 14 days. If longer human administration is planned, subchronic studies must last 6 to 24 months (17).
Animals are observed for behavior and weight changes, eating habits, and general appearance. Full-blood analyses are performed, as well as electrocardiograms and ophthalmologic examinations. The animals are eventually killed, and all organs are closely scrutinized. Cause of death is sought in any animals that die before testing is completed. Mutagenicity by means of the Ames text and carcinogenicity in two species are determined. The full reproductive cycle is observed and analyzed—from production of eggs and sperm; through conception, delivery, and growth; to sexual maturity of the offspring (16). Preclinical testing for potential teratogenicity may be misleading, as animals may have different sensitivities than humans or may produce different metabolites. In addition, animals are exposed to much higher doses than those that will be used clinically in humans (18). Despite the uncertainty about the clinical relevance of these tests, they are used to determine the pregnancy safety labeling when a new drug is brought to market. Unique characteristics of some compounds will mandate specific studies—for example, additional toxicologic assays for NMDA-receptor blockers, which can produce neuronal death (19). On initial testing, vigabatrin was noted to produce vacuolization of central nervous system white matter in dogs and rats (2,20). Human testing was halted until, among other things, long-term studies could be performed in nonhuman primates. Fortunately, no evidence of similar toxicity was found either in primate nonhuman species or in man. Some agents are being closely scrutinized for their effects on the retina, as a result of unexpected visual changes that were associated with the GABA-antagonist vigabatrin (21). Additionally, some agents produce active metabolites, which may produce independent efficacy and toxicity. These compounds must undergo the same rigorous testing as the parent compound.
The potential of a compound to produce even frequent idiosyncratic reactions is unlikely to be revealed during preclinical testing. Moreover, metabolic pathways in humans may differ from those in other species, leading to unexpected toxic reactions. Physicians must not mistakenly believe that the TI, which assesses only dose-related neurotoxicity, indicates overall safety. For example, despite a high TI, after it was marketed, felbamate was found to be associated with a risk of inducing hematologic and hepatic disorders (22).
TESTING IN HUMANS
Once preclinical testing has been completed, human investigation of the agent, consisting of four phases, can begin.
Phase 1
During phase 1 testing, a compound is used for the first time in humans, usually healthy volunteers. Single, increasing doses are followed by long-term administration of the agent. The dose at which toxicity first appears is assessed, as well as any dose-related adverse events and the maximum tolerated dose (MTD). Half-life, time to maximum concentration, clearance, presence or absence of active metabolites, and route of elimination are all determined (23). In total, fewer than 100 normal individuals will be exposed to the agent. Phase 1 testing of AEDs usually includes patients as volunteers—a crucial point, as the MTD may be different in patients with epilepsy. In addition, most AEDs are used initially as adjunctive therapy, and drug-drug interactions should be known before efficacy testing begins.
Appropriate testing during phase 1 is critical for all phases that follow. Effective agents may appear to be ineffective if inadequate doses are administered. Conversely, selection of higher-than-optimal doses may result in an agent appearing overly toxic or being associated with excessive dropouts during clinical trials. Trial designs for efficacy testing may also be influenced by characteristics revealed during phase 1 testing.
Phases 2 and 3
During phase 2 trials, initial clinical trials determine the efficacy and toxicity of an agent. The first efficacy trial is usually termed proof of principle. This trial is used to obtain an initial signal of efficacy, and may be used by the sponsor of a drug to make a go/no-go business decision about continued development of the agent. Proof-of-principle trials are often open-label in design; even if they are placebo controlled, such trials may not be powered to detect a statistically significant difference. Design of the proof-of-principle trial is important, as there is great danger of discarding a potentially useful agent (24). In phase 3 trials, efficacy and safety assessments continue and may include evaluation of specialized populations, such as children, or specific seizure syndromes.
Two “adequate and well-controlled clinical trials”—so-called pivotal trials—that demonstrate efficacy must be conducted to obtain FDA approval (17). Long-term safety studies must also be completed. Of the several thousand patients who will be exposed to an AED during phases 2 and 3, several hundred will have multiple years of exposure. When these phases are complete, the sponsoring pharmaceutical company will file a New Drug Application (NDA). This document, which contains all the information obtained in preclinical and clinical testing, forms the basis on which the FDA renders its decision.
Phase 4
Once a drug is approved, phase 4, or postmarketing, testing begins, which includes surveillance studies, studies for new indications or expanded populations, and large, less tightly regulated trials.
EFFICACY AND SAFETY TRIALS
Some characteristics of patients with epilepsy and of the agents used to treat the disease make well-controlled trials very difficult to conduct. The following sections outline some major issues in the design and implementation of such trials.
Selection of Population
Epilepsy Syndrome
The term antiepileptic drug implies a single disease. However, epilepsy comprises a diverse group of syndromes, each with a unique clinical presentation and often with different genetics, etiology, and possibly underlying biochemical defect (25). It would be astounding if a single agent had equal efficacy in all these syndromes. In fact, most AEDs are effective either in certain seizure types or in specific seizure syndromes. As the most common seizure type in adults, partial seizures are frequently chosen for study in pivotal trials. As a result, many AEDs are approved for the treatment of partial seizures and comparatively few for other seizure types, such as absence, myoclonus, and infantile spasms. Trials in other populations have become more common. For example, lamotrigine received approval for use in seizures associated with the Lennox-Gastaut syndrome based on positive clinical trials (26). Topiramate was shown to reduce the number of seizures in a trial of patients with Lennox-Gastaut syndrome (27), as well as in a novel clinical trial of patients with primary generalized tonic-clonic convulsions (28). To date, large-scale randomized trials of the new agents have not been conducted in patients with absence or myoclonic epilepsy.
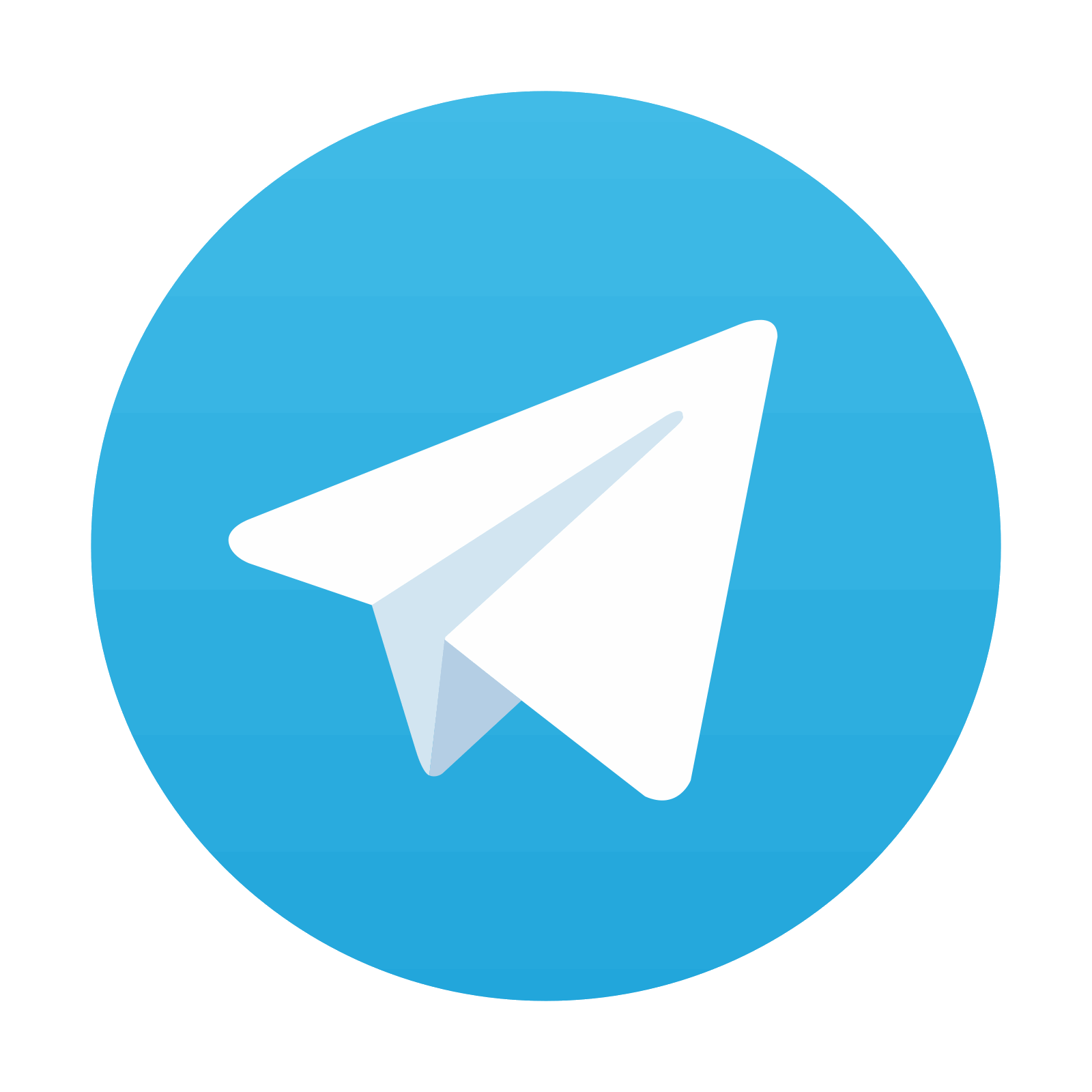
Stay updated, free articles. Join our Telegram channel
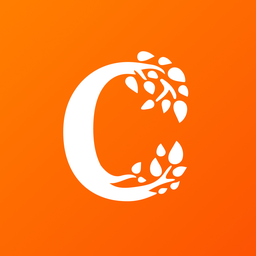
Full access? Get Clinical Tree
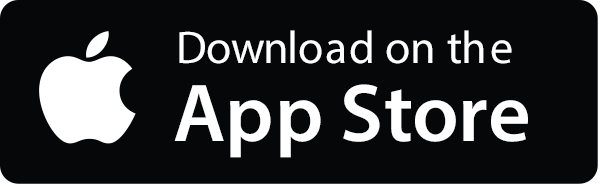
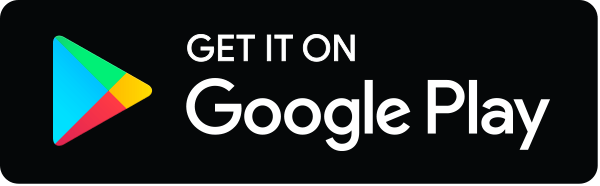
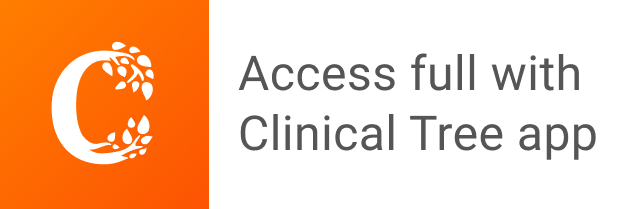