The common designation of a group of neurotransmitters as purines is a misnomer; what are called purinergic signaling molecules are the nucleoside and nucleotide derivatives of purine and perhaps pyrimidine bases.
The two principal purinergic signaling molecules are adenosine and adenosine triphosphate (ATP). ATP is stored in small synaptic vesicles and released in a Ca2+-dependent fashion, whereas adenosine is released from nonvesicular cytoplasmic stores, likely via bidirectional nucleoside transporters.
Purine receptors form a relatively large and diverse group and have been categorized as P1 and P2 receptors.
P1 receptors, also called adenosine receptors, bind adenosine and its analogs and are G protein–coupled. Stimulant drugs of the methylxanthine family, including caffeine, are antagonists of adenosine receptors. P2 receptors consist of both ligand-gated ion channels termed P2X receptors and G protein–coupled receptors termed P2Y receptors. P2X receptors play an important role in pain processing.
Cannabinoids, the principal active ingredients of marijuana, primarily act in the brain on the CB1 receptor, a G protein–coupled receptor found on presynaptic terminals in the central nervous system (CNS).
Anandamide and 2-arachidonylglycerol are endogenous cannabinoids (endocannabinoids), which are released from postsynaptic cells and activate presynaptic CB1 receptors.
Nitric oxide (NO) is generated from arginine by NO synthase, which is stimulated by activation of postsynaptic NMDA receptors and increases in cellular Ca2+ levels. It diffuses out of cells and activates soluble guanylyl cyclase leading to the production of cGMP in adjacent cells and nerve terminals.
Carbon monoxide (CO) is produced by the breakdown of heme by heme oxygenase-2 and also may function as an atypical, diffusible messenger. Hydrogen sulfide (H2S) is another “gas transmitter”: it is generated from cyst(e)ine by the enzyme cystathionine β-synthase.
Neurotrophic factors are polypeptides or small proteins that support the growth, differentiation, and survival of neurons. They produce their effects by activation of tyrosine kinases.
The neurotrophins, which comprise nerve growth factor (NGF), brain-derived neurotrophic factor (BDNF), and neurotrophin-3 (NT-3) and neurotrophin-4 (NT-4), act by binding to a family of tropomyosin receptor kinase (Trk) receptors, TrkA, TrkB, and TrkC, with intrinsic tyrosine kinase activity.
Numerous other growth factors, such as glial cell line–derived neurotrophic factor (GDNF), vascular endothelial growth factor (VEGF), and neuregulin, are important in regulating the nervous system.
Several cytokine-like factors, including ciliary neurotrophic factor (CNTF) and interleukin-6 (IL-6), are characterized by binding to receptors that activate a family of protein tyrosine kinases called Janus kinases (JAKs), which in turn activate transcription factors called signal transducers and activators of transcription (STATs).
Many additional cytokines, best understood for their role in the immune system and inflammatory responses, also are important in the regulation of CNS function. Prominent examples include interleukin-1 (IL-1), tumor necrosis factor-α (TNF-α), and transforming growth factor-β (TGF-β).
Chemokines are small proteins involved in immune responses; in the brain, chemokines are expressed predominately by microglia.
Atypical neurotransmitters include a host of intercellular signaling molecules that have unusual properties or more recent dates of discovery compared with better known small-molecule and neuropeptide neurotransmitters that were reviewed in Chapters 5 to 7. The atypical neurotransmitters include the purinergic neurotransmitters adenosine and adenosine triphosphate (ATP), endogenous cannabinoids (endocannabinoids), the gases nitric oxide (NO) and carbon monoxide (CO), and families of neurotrophic factors and cytokines. Each of these neurotransmitters is found in specific subsets of neurons (and in some cases glia), has specific biochemic machinery for its synthesis, and exerts its biologic effects via activation of specific receptors or enzymes. Because of their discrete anatomic distributions and, in many cases, modulatory functions, these atypical neurotransmitters, their receptors, and the proteins involved in their production and degradation are potentially significant targets for drug development. Not discussed in this chapter are transient receptor potential (TRP) channels, which are covered in Chapters 2 and 11. Many of these channels respond to a host of endogenous molecules (eg, lipids, nucleotides, inorganic ions) or environmental signals (eg, temperature, mechanical stress) and thus function analogously to other signaling mechanisms presented in this chapter.
The purinergic neurotransmitters, adenosine and ATP, were long considered improbable mediators of neurotransmission because of their roles in intermediary metabolism and as building blocks of RNA and DNA. However, we now know that they are concentrated at certain synapses, they are released in response to synaptic stimulation, and they activate specific receptors to produce significant responses in target neurons. It is also established that purinergic receptors mediate the actions of several pharmacologic agents, most notably caffeine and related stimulants.
The practice of referring to these signaling molecules as purines is to some degree a misnomer. Purines are ring-structured basic compounds that include adenine and guanine. These molecules do not function as neurotransmitters, nor do the pyrimidines, which include uracil, thymidine, and cytosine. So-called purinergic signaling molecules are in fact the nucleoside and nucleotide derivatives of purine bases 8–1A. The two principal neurotransmitters in this family are adenosine and ATP. Related to these are the adenine dinucleotides, which consist of two adenosine molecules covalently linked by a chain of two to six phosphate groups. Adenine dinucleotides are represented by the abbreviation ApnA, wherein n equals the number of phosphates between the two adenosines 8–1B. ApnA molecules are released by neurons and thus may be considered part of the purinergic signaling family. There is also some evidence that nucleoside and nucleotide derivatives of pyrimidine bases may serve as neurotransmitters, although this possibility remains poorly characterized.
8–1
Structures of purinergic compounds. A. Purines, such as adenine, are basic ring-structured molecules, often referred to as bases. Nucleosides are molecules composed of a pentose sugar, such as ribose, covalently linked to a nitrogen atom in the base. Nucleotides are nucleosides whose monophosphate, diphosphate, or triphosphate groups are linked to the 5´ carbon of a pentose sugar. Adenine’s nucleosides and nucleotides are neurotransmitters, but adenine is not involved in neurotransmission. B. Adenine dinucleotides are two nucleosides linked by two to six phosphate groups. For example, Ap4A signifies a diadenosine molecule with four intervening phosphate groups.

Despite their similarities, adenosine and ATP have distinct properties. ATP and ApnA are stored in small synaptic vesicles and are released in response to action potentials in a Ca2+-dependent process similar to the release process for classic neurotransmitters (Chapter 3). Indeed, ATP and classic neurotransmitters often can be detected in the same synapses and even in the same synaptic vesicles indicating that they can be coreleased. Release of ATP from sympathetic nerve terminals as well as vascular endothelial cells and tumor cells may play a particularly important role in various pain states. In contrast, adenosine is released from nonvesicular cytoplasmic stores and most likely reaches the extracellular space by one of two means. First, any of several bidirectional nucleoside transporters can secrete adenosine into the extracellular space, including the synapse. Second, ATP is very rapidly metabolized into adenosine once it is released from a cell. A membrane-bound ectodiphosphohydrolase converts ATP into ADP and AMP, and subsequently a membrane-associated or soluble ecto-5´-nucleotidase converts AMP into adenosine. Because the conversion of ATP to adenosine takes place in less than a second, the synaptic release of ATP should be regarded as an important source of extracellular adenosine.
The schematic representation of the purinergic synapse in 8–2 shows the release of ATP leading to the production of other purinergic compounds and to the subsequent activation of several types of purinergic receptors. It should be noted that ApnA is hydrolyzed much more slowly than ATP and can reside in the synaptic cleft for longer periods of time.
8–2
A purinergic synapse. Adenosine triphosphate (ATP) and ApnA typically are colocalized with a classic neurotransmitter and are released into the synaptic cleft in a Ca2+-dependent fashion. After it is released, ATP can directly activate P2Y and P2X receptors. P2Y receptors are coupled to G proteins and activate second messenger systems. Most are coupled to Gq/11 and activate phospholipase C (PLC) and the phosphatidylinositol pathway. P2X receptors are ligand-gated channels that depolarize the postsynaptic membrane. ATP remaining in the synapse is rapidly converted into adenosine (Ado) by the actions of an ectodiphosphohydrolase and an ecto-5´-nucleotidase. Subsequently, Ado is able to activate presynaptic and postsynaptic G protein–coupled P1 receptors (A1 and A2) and regulate adenylyl cyclase (AC) and the cAMP pathway, and in turn can be recycled into the presynaptic cell by means of a Na+-dependent transporter (N1).

Nucleoside transporters are membrane-bound proteins that shuttle purine and pyrimidine nucleosides into and out of many cells, including neurons. The transporters are purine-selective or pyrimidine-selective. Some act to concentrate the nucleosides in a cell in a Na+-dependent fashion. Others transport nucleosides down their concentration gradients. Pharmacologic and genetic studies indicate that there are at least seven nucleoside transporters, which belong to two gene families. The so-called equilibrative nucleoside transporter gene family (Slc29A1–A4 or ENT1–4), which consists of four subtypes (Slc29A1 or ENT1–4), mediates both efflux and influx of nucleosides; the concentrative nucleoside transporter family, which has three members (Slc28A1–A3 or CNT1–3), mediates Na+-dependent influx of nucleosides. Although much remains to be learned about the structure and function of these proteins, they have been exploited in the development of powerful therapeutic agents. A number of cancer chemotherapeutic drugs, such as gemcitabine, and several potent antiviral compounds, such as zidovudine (AZT), which are used in the treatment of AIDS, are nucleoside analogs that enter target cells by means of nucleoside transporters. Given the powerful effects that adenosine exerts on brain function, it is possible that drugs that regulate nucleoside transporters might eventually prove useful in the treatment of neuropsychiatric disorders.
Purine receptors form a relatively large and diverse group of proteins that comprise two main subgroups, referred to as P1 and P2 receptors. Characteristics of both of these receptor families are discussed here and are summarized in 8–1. P1 receptors, also known as adenosine receptors (A1 and A2), bind adenosine and its analogs and are coupled to G proteins. Four subtypes have been identified (A1, A2A, A2B, A3), and each displays the canonical seven-transmembrane domains found in other members of the G protein–coupled receptor superfamily. The A1 receptor subtype is the most widely expressed in the brain and spinal cord and has the highest affinity for adenosine. Its activation has been implicated in the putative anxiolytic, anticonvulsant, analgesic, and sedative properties of adenosine. Conversely, antagonism of A1 receptors by methylxanthines such as caffeine and similar drugs results in stimulatory effects, including increased alertness at low doses and anxiety and irritability at much higher doses (8–1; see also Chapter 13).
Receptor Subtype | ||||
|
|
|
|
|
|
| |||
|
|
8–1 Java Nation
Although most people have never heard of the term methylxanthines, almost everyone is familiar with several of these drugs and their behavioral effects. One of these agents, 1,3,7-trimethylxanthine, is better known to most of us as caffeine, arguably the world’s most widely used psychoactive substance as it and its derivatives—including theophylline (found in tea) and theobromine (found in cocoa)—are found in coffee, tea, cocoa, chocolate, most energy drinks, and many soft drinks. The stimulatory properties of these drugs are caused primarily by the antagonism of the adenosine A1 receptor, but also to some degree the A2A receptor (Chapter 13). The average daily dose of caffeine in the United States is approximately 200 mg, which is equivalent to two cups of coffee or approximately four cans of cola. At such doses in most individuals, it decreases fatigue, can enhance cognition, and does not produce significant side effects. However, prolonged use of products containing methylxanthines can lead to tolerance to the stimulatory properties of the drug and to physical dependence—which is not synonymous with addiction (Chapter 16). As anyone who habitually consumes large volumes of coffee knows, quitting the habit suddenly can lead to withdrawal symptoms that include headaches, drowsiness, and nausea. In addition, consuming high doses of caffeine (400 mg or more per day) can lead to anxiety and nervousness and can trigger panic symptoms in vulnerable individuals.
The two subtypes of the A2 receptor, A2A and A2B, have a somewhat lower affinity for adenosine than do A1 receptors. The A2B receptor is ubiquitous in the human body yet is expressed only at very low levels in the brain and spinal cord. In contrast, the A2A receptor is highly concentrated in the dorsal striatum, nucleus accumbens, and olfactory tubercle—three brain regions that receive rich dopaminergic innervation (Chapters 6, 16–18). Indeed, the actions of adenosine and dopamine are interrelated in these regions. In the striatum, A2A receptor agonists inhibit dopamine D2 receptor–mediated behaviors, and A2A antagonists mimic D2 receptor agonists. The latter action likely contributes to the locomotor-activating properties of methylxanthines. The inverse relationship between adenosine and dopamine has led to speculation that selective A2A antagonists might be useful in the treatment of Parkinson disease, as discussed in the next section of this chapter.
The A3 receptor is expressed at low levels in the brain, and its function is not yet known. It is distinguished from the other subtypes in that it has a much lower affinity for adenosine. Whereas A1 and A2 receptors bind adenosine with nanomolar affinity, micromolar concentrations are necessary to activate A3 receptors.
The P2 receptor family is intriguing because it comprises both G protein–coupled receptors (the P2Y family) and ligand-gated ion channels (the P2X family). Eight human P2Y receptor subtypes have been cloned and characterized. These receptors display unique pharmacologic profiles, and bind purine and pyrimidine nucleotide diphosphates and triphosphates, as well as ApnA molecules, with varying affinities 8–1. The P2Y1 receptor, for example, binds ATP and ADP but not UTP or UDP. In contrast, the P2Y2 receptor is activated by ATP and UTP with equal affinity. The regional distribution and functional significance of P2Y receptors in the central nervous system (CNS) are still being characterized.
Seven P2X receptor subtypes are known. Each is an ATP- or ApnA-gated cation channel composed of multiple subunits. Although the exact stoichiometry of P2X receptors is unknown, functional homomeric receptors have been constituted in vitro, and there is evidence for heteromeric P2X2/3 receptors. Because these receptors have multiple subunits, each of the cloned proteins in 8–1 should be regarded as a subunit rather than as a complete receptor. P2X receptor activation leads to rapid Na+, K+, and Ca2+ flux and subsequent membrane depolarization. These fast-acting channels have been detected in the peripheral nervous system, neuromuscular junction, spinal cord, and many brain regions. When on postsynaptic cells, their activation leads to membrane depolarization. They are also found on many presynaptic nerve terminals throughout the CNS, and their activation routinely leads to enhancement of transmitter release. Via their actions in both the periphery and the spinal cord, they appear to play important roles in mediating a variety of different forms of pain and thus are important targets for the development of novel analgesic medications (Chapter 11).
The cloning of the P1 and P2 receptor families opened up numerous avenues for investigating purine function in the nervous system. Receptor-selective agonists and antagonists are being developed, and transgenic animals that underexpress or overexpress individual receptor subtypes have been generated. Although many putative roles for nucleosides and nucleotides have been hypothesized, only a handful have been clearly established in the CNS.
Adenosine has both anxiolytic and hypnotic properties, and the administration of adenosine receptor antagonists confirms these observations. As described in 8–1, caffeine and other methylxanthine compounds, by blocking endogenous adenosine action, increase alertness and improve cognitive performance. The cognition-enhancing properties of these drugs are particularly interesting, partly because of the wide use of caffeine, and partly because adenosine receptor antagonists may prove useful as symptomatic treatments for the cognitive deficits associated with Alzheimer disease and other forms of dementia (Chapter 18).
Adenosine also is being investigated for its neuroprotective effects; it is hoped that these properties might be exploited in the treatment of stroke. Stroke is characterized, in part, by an ischemia-induced increase in glutamate levels followed by an overstimulation of glutamate receptors and massive influx of Ca2+ into neurons, which unleashes a cascade of cytotoxic events (Chapter 20). Thus, an ideal neuroprotective agent would inhibit glutamate release presynaptically and prevent postsynaptic membrane depolarization and subsequent Ca2+ influx. Adenosine can accomplish both feats. Adenosine activation of presynaptic A1 receptors inhibits the release of glutamate and other neurotransmitters at many synapses throughout the brain. Activation of postsynaptic A1 receptors opens K+ channels, and thereby hyperpolarizes neurons and counters the excitatory effects of glutamate. The net result is believed to be decreased Ca2+ influx and decreased neuronal death. Experiments in animal models support this hypothesis. The administration of selective A1 agonists just before or during an ischemic event in animals significantly reduces neuronal loss. Conversely, the administration of A1 antagonists augments ischemic brain damage. It is important to note that ischemia dramatically increases adenosine levels in the brain that will likely influence the net effects of drugs that target adenosine receptors.
Although activation of A1 receptors may provide some measure of neuroprotection, the actions of adenosine at other receptor subtypes may exert the opposite effect. Activation of A2 receptors, for example, has had deleterious effects on animals in models of stroke. Conversely, A2-selective antagonists can produce neuroprotective effects. Acute activation of A3 receptors with selective agonists also profoundly increases brain damage and mortality in animal models. In contrast, chronic administration of these adenosine agonists improves survival. Adenosine’s actions on vascular tone and platelet function likely contribute to these various observations. The interplay that occurs among adenosine receptor subtypes during ischemia clearly requires further investigation.
Adenosine systems also may prove to be promising targets for drugs designed to treat Parkinson disease. As discussed in Chapter 18, this disease is caused by a loss of dopaminergic innervation to the striatum, which leads to rigidity, tremor, and slowness of movement. The striatum, as previously mentioned, expresses high levels of adenosine A2A receptors. A2A receptor agonists produce biochemical and behavioral effects (decreased motor activity) that mimic those associated with Parkinson disease because they decrease dopamine neurotransmission in the striatum. Accordingly, blocking A2A receptors might have the opposite effect; that is, dopamine function in the striatum and motor activity might be expected to increase.
Several orally active compounds that selectively block A2A receptors have been described. When used in animal models of Parkinson disease, these drugs significantly reverse motor deficits while inducing little, if any, dyskinesia, a side effect associated with L-dopa therapy (Chapter 18). Moreover, when coadministered with L-dopa, A2A antagonists diminish L-dopa-induced dyskinesia. Currently, A2A receptor antagonists are in development for the treatment of Parkinson disease.
Adenosine also has been reported to exhibit anticonvulsant, anxiolytic, and antidepressant activity, as well as both analgesic and pain-enhancing properties. Although these purported functions require further investigation, they point to the therapeutic potential of selective adenosine receptor agonists and antagonists.
Over the last decade, a role for ATP and P2X receptors in pain has become apparent. Application of ATP to human skin elicits acute pain. Several different subtypes of P2X receptors are found in sensory neurons in dorsal root ganglia and other sensory ganglia with P2X3 and P2X2/3 receptors generally having the highest levels of expression. These receptors are also found at nociceptive nerve terminals in the periphery where they can respond to the release of ATP from various sources including tumors and vascular endothelium 8–3.
8–3
Role of P2X receptors in pain processing. Hypothetical scheme of the actions of purine nucleotides and nucleosides in pain pathways. At sensory nerve terminals in the periphery, P2X3 homomeric and P2X2/3 heteromeric receptors have been identified as the principal P2X receptors present, although recent studies have also shown expression of P2Y1 and possibly P2Y2 receptors on a subpopulation of P2X3 receptor-immunopositive fibers. Other known P2X receptor subtypes (1–7) are also expressed at low levels in dorsal root ganglia. Although less potent than ATP, adenosine appears to act on sensory terminals, probably directly via P1(A2) receptors; however, it also acts synergistically to potentiate P2X2/3 receptor activation, which may be true for serotonin, capsaicin, and protons as well. At synapses in sensory pathways in the CNS, ATP acts postsynaptically via P2X2, P2X4, and/or P2X6 receptor subtypes, perhaps as heteromultimers. As well, after release, ATP is rapidly degraded into adenosine, which acts as a prejunctional inhibitor of neurotransmission via P1(A2) receptors. P2X3 receptors on the central projections of primary afferent neurons in lamina II of the dorsal horn mediate facilitation of glutamate and probably also ATP release. Sources of ATP acting on P2X3 and P2X2/3 receptors on sensory terminals include sympathetic nerves, endothelial, Merkel, and tumor cells. Yellow dots, molecules of ATP.

Molecular and pharmacologic studies of P2X receptors, in particular P2X3 and P2X2/3 receptors, suggest that while they may not be critically involved in acute pain sensation, changes in their levels and functions importantly contribute to the development and maintenance of various forms of neuropathic and inflammatory pain. As these pain states are common, and among the most resistant to treatment, P2X receptors appear to be important targets for potential novel analgesic medications (Chapter 11).
In 1964, identification of the psychoactive ingredient in marijuana as Δ9-tetrahydrocannabinol (THC) led to the synthesis of related compounds that displayed saturable binding and pharmacology consistent with the existence of a so-called cannabinoid receptor. Some of these agents 8–4 were found to cause a GTP-dependent inhibition of adenylyl cyclase, which suggested that the receptor for cannabinoids is a member of the large superfamily of G protein–coupled receptors. These observations led to the eventual cloning of two cannabinoid receptors known as the CB1 and CB2 receptors. CB1 receptors are found at very high concentrations throughout the brain including the cerebellum, hippocampus, basal ganglia, cortex, brainstem, thalamus, and hypothalamus. This broad anatomic distribution of the CB1 receptor helps to explain the diverse effects elicited by marijuana 8–2. The CB2 receptor has low overall homology with CB1 and is found primarily in immune cells with some limited expression in the brain.
8–2 Marijuana
Marijuana is the most commonly used illegal drug in the United States and in many other countries throughout the world. The term marijuana refers to a preparation that is typically smoked and made from the dried leaves of the hemp plant Cannabis sativa (hence the name cannabinoids). Several other preparations are commonly used, including hashish, which consists of the psychoactive resin pressed into blocks, and bhang, a liquid distillate used in India. Throughout much of its history, cannabis has been known as much for its analgesic properties as its psychoactive effects. However, controversy continues to surround its use as an analgesic and for other medical indications. Although it is used to stimulate appetite in patients with AIDS, to reduce intraocular pressure in patients with glaucoma, and to treat the nausea caused by cancer chemotherapy, debate about the abuse potential of “medical marijuana” continues.
The marijuana intoxication syndrome varies greatly. Some individuals become giddy, and others become morose and depressed. Use of the drug also may be accompanied by introspection and a sense of time moving slowly. In many ways, marijuana intoxication is similar to intoxication produced by hallucinogens; indeed cannabinoids often are considered “minor hallucinogens.” Distortions of sensory perceptions are fairly common during marijuana intoxication, and hallucinations may occur, albeit rarely. Potential adverse effects include an impairment in short-term and perhaps long-term memory, and impaired motor coordination. The addicting properties of marijuana are covered in Chapter 16.
CB1 and CB2 receptors couple to effectors by means of Gi/o proteins. Like other receptors that couple with these G proteins, CB1 receptors inhibit adenylyl cyclase and, depending on the cell type, inhibit voltage-gated Ca2+ channels or stimulate inwardly rectifying K+ channels. As they are primarily found on presynaptic terminals of both excitatory and inhibitory neurons, activation of CB1 receptors inhibits transmitter release and thereby synaptic transmission in a wide range of brain regions.
The identification of cannabinoid receptors led to the speculation that an endogenous cannabinoid-like substance might exist; such speculation was partly based on the discovery of endogenous opioid peptides soon after the detection of opioid receptors (Chapter 7). Because cannabinoids are highly lipophilic, the search for an endogenous substance focused on membrane extracts from the brain. This led to the identification of two substances, anandamide and 2-arachidonylglycerol (2-AG), which function as endogenous cannabinoids or endocannabinoids: they are released in response to neural activity and activate CB1 receptors. As shown in 8–5A, both anandamide and 2-AG are thought to be synthesized from membrane-derived lipid precursors. Abundant evidence suggests that their release from postsynaptic neurons is coupled to their synthesis. Specifically, in a variety of cell types, strong depolarization leads to Ca2+ influx via voltage-dependent Ca2+ channels and this in turn causes activation of the enzymes responsible for generating anandamide or 2-AG 8–6. In certain cell types, the generation of these endocannabinoids can also be stimulated by activation of postsynaptic G protein–coupled receptors, specifically Gq-linked metabotropic glutamate receptors or muscarinic receptors 8–6.
8–6
Endocannabinoids function as retrograde messengers to inhibit the presynaptic release of neurotransmitter. Strong activation of voltage-dependent Ca2+ channels, metabotropic glutamate receptors (mGluR), or muscarinic acetylcholine receptors (mAChR) in the postsynaptic cell can cause the production of endocannabinoids. These can escape from the postsynaptic cell and activate presynaptic CB1 receptors, which inhibit neurotransmitter release.
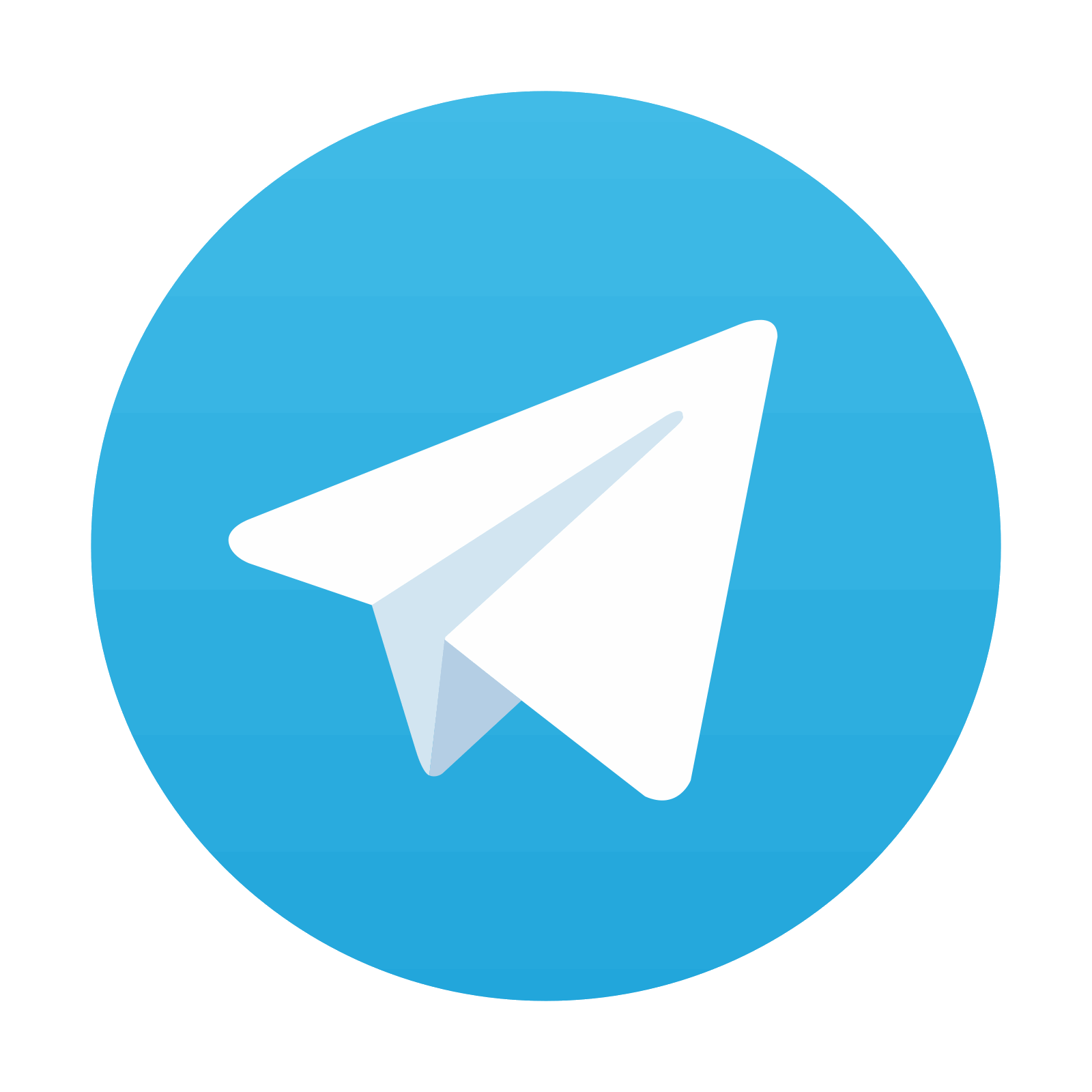
Stay updated, free articles. Join our Telegram channel
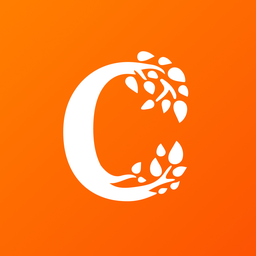
Full access? Get Clinical Tree
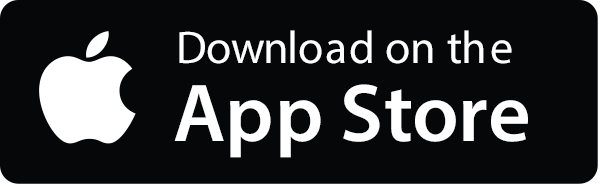
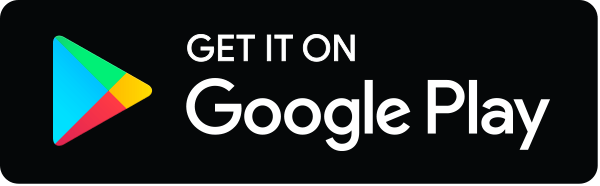
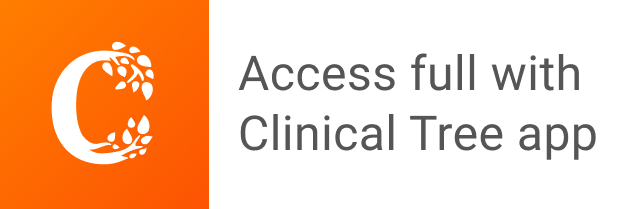