An understanding of drug action in the brain must integrate knowledge of the molecular and cellular actions of a drug with their effects on brain circuitry.
The clinical actions of a drug in the brain often are due to neural plasticity—the long-term adaptations of neurons to the sustained short-term actions of a drug.
The binding of a drug to its specific target(s) normally is saturable and stereoselective.
The specific binding of a drug to its target is quantified according to its affinity for the target, expressed as a dissociation constant (Kd), and the total amount of binding (Bmax).
Potency of a drug describes the strength of binding between the drug and its target; efficacy describes the maximal biologic effects that the drug exerts by binding to its target.
Drugs can be classified as agonists, partial agonists, inverse agonists, partial inverse agonists, or antagonists.
Modern neuropharmacology takes advantage of the tools of molecular biology, genetics, and cell biology as well as combinatorial chemistry, which is used to generate novel molecules that may function as new drugs.
Functional genomics and proteomics will help identify novel drug targets.
Pharmacogenetics will guide the choice of drug treatments based on an individual’s genetic constitution.
Neuropharmacology is the scientific study of the effects of drugs on the nervous system. Its primary focus is the actions of medications for psychiatric and neurologic disorders as well as those of drugs of abuse. Neuropharmacology also uses drugs as tools to form a better understanding of normal nervous system functioning. The goal of neuropharmacology is to apply information about drugs and their mechanisms of action to develop safer, more effective treatments and eventually curative and preventive measures for a host of nervous system abnormalities. The importance of neuropharmacology to medical practice, and to society at large, is difficult to overstate. Drugs that act on the nervous system, including antidepressant, antianxiety, anticonvulsant, and antipsychotic agents, are among the most widely prescribed medications. Moreover, commonly prescribed medications that act on other organ systems often are associated with side effects that involve the nervous system and in turn may limit their clinical utility. In addition, a substantial number of individuals use common substances, such as caffeine, alcohol, and nicotine, that are included in the domain of neuropharmacology because of their effects on the central nervous system (CNS). In a much smaller fraction of the population, these and other drugs are used compulsively, in a manner that constitutes an addiction. Drug abuse and addiction exact an astoundingly high financial and human toll on society through direct adverse effects, such as lung cancer and hepatic cirrhosis, and indirect adverse effects—for example, accidents and AIDS—on health and productivity. Still other common afflictions of the nervous system, such as Alzheimer disease as just one example, are awaiting effective medications, further emphasizing the importance of neuropharmacology.
Neuropsychopharmacology is an all-encompassing term that typically is applied to all types of drug effects that influence nervous system functioning. The term psychopharmacology is often used to describe the effect of drugs on psychologic parameters such as emotions and cognition. Drugs that influence behavior are known as psychotropic agents. In this book we use the term neuropharmacology to describe the study of all drugs that affect the nervous system, whether they affect sensory perception, motor function, seizure activity, mood, higher cognitive function, or other forms of nervous system functioning.
The actions of drugs that affect the nervous system are considerably more complicated than those of drugs that act on other organ systems. To understand how drugs act on the nervous system, it is critical to integrate information about the molecular and cellular actions of a drug with knowledge of how these actions affect brain circuitry—a circuitry that is constantly changing in structure and function in response to both pharmacologic and nonpharmacologic input from the environment. The complexity that underlies such actions can be illustrated by consideration of fluoxetine, a widely prescribed antidepressant, and furosemide, a widely prescribed diuretic. The chemical actions of these drugs are fairly simple. Both drugs initially bind to their specific protein target: fluoxetine binds to and inhibits serotonin transporters, which normally inactivate the actions of the neurotransmitter serotonin (Chapter 6), and furosemide binds to and inhibits Cl− channels located in the ascending loop of Henle in nephrons of the kidney. However, the relation between the chemical and clinical actions of these drugs—particularly those of fluoxetine—requires a more elaborate explanation.
The association between furosemide’s chemical and clinical activity is relatively straightforward. By inhibiting Cl− transport in Henle’s loop, furosemide causes more Cl− to remain in the lumen of the nephron tubule, which in turn requires more H2O to remain in the tubule. Furosemide exerts this same effect on all nephrons in the kidney, and the increase in H2O in individual nephron tubules combines to cause diuresis at the level of the kidney. Diuresis is achieved as soon as effective concentrations of the drug reach the kidney’s extracellular fluid, and is maintained with repeated use of the drug—for example, in the treatment of chronic congestive heart failure.
The relationship between the chemical and clinical actions of fluoxetine is more intricate and also more speculative. Most drugs that act on the nervous system interact with only the minute subset of the brain’s neurons that express the initial protein target of the drug. Fluoxetine directly affects only those neurons that use serotonin as a neurotransmitter—a few 100,000 out of approximately 100 billion neurons in the brain. By inhibiting serotonin reuptake by these neurons, fluoxetine enhances serotonergic transmission throughout the brain, but it is not known with certainty where in the brain enhanced serotonin function causes an antidepressant effect. Similarly, little is known about which of serotonin’s 14 known receptors must be activated to achieve an antidepressant response. Moreover, the mood-elevating effects of fluoxetine are not evident after initial exposure to the drug but require its continued use for several weeks. This delayed effect suggests that it is not the inhibition of serotonin transporters per se, but some adaptation to sustained increases in serotonin function that mediates the clinical actions of fluoxetine. However, where these adaptations occur in the brain and the nature of the adaptations at the molecular level have yet to be identified definitively.
The clinical actions of fluoxetine, like those of many neuropharmacologic agents, reflect drug-induced neural plasticity, which is the process by which neurons adapt over time in response to chronic disturbance. Consequently, to understand fully the effects of a neuropharmacologic drug, we must determine not only the initial effects of the drug but also the intraneuronal signals that control a neuron’s adaptations over time, the interneuronal signals through which neurons communicate with one another, and the ways in which large groups of neurons operate in circuits to produce complex brain functions.
Parts I and II of this book explore the intraneuronal and interneuronal signals that enable communication among neurons, which are fairly well understood. Part III addresses the relationships between circuits of neurons and complex brain functions, about which much remains to be discovered.
Neuropharmacology has contributed to many important advances in the neurosciences during the past several decades. Drugs have been used as tools to dissect the functions of the brain and of individual nerve cells under normal and pathophysiologic conditions. Historically, neuropharmacology has involved the delineation of diverse molecules that function as neurotransmitters in the nervous system, including monoamines, amino acids, purines, and peptides. The identification of many of these neurotransmitters and the elucidation of their synthesis, degradation, and receptors occurred in conjunction with studies of synthetic and plant substances that were known to exert profound effects on behavior. The neuropharmacology of ergot alkaloids, cocaine, and reserpine, for example, led to the discovery and characterization of monoamine neurotransmitter systems; opiate alkaloids such as morphine led to endogenous opioid systems; nicotine, muscarine, and cholinesterase inhibitors led to cholinergic systems; and caffeine and related substances led to purinergic systems.
Neuropharmacology also played a fundamental role in the delineation of the numerous receptor subtypes through which neurotransmitters elicit biologic responses. The early idea that one neurotransmitter acts on only one receptor was replaced decades ago with the recognition that for each neurotransmitter there are multiple receptors. This discovery led to the development of synthetic drugs with increasing selectivity for individual types of receptors, and the evolution of these neuropharmacologic agents has represented important advances in clinical medicine. These advances include the use of selective β1-adrenergic antagonists for cardiovascular disease, selective β2-adrenergic agonists for asthma, μ-opioid antagonists for opiate overdose, and 5HT1D-serotonin agonists for migraine, to name just a few examples.
As well, the identification of multiple receptor subtypes for neurotransmitters contributed to the recognition of complex postreceptor signal transduction cascades through which receptors ultimately produce their biologic responses. From G proteins to second messengers to protein phosphorylation pathways to regulation of gene expression, studies of the effects of drugs on the nervous system have provided crucial windows onto the functioning of intracellular signaling. For instance, investigation of the mechanisms by which organic nitrates cause vasodilation in the treatment of angina led to the discovery of nitric oxide as a critical signaling molecule, and studies of aspirin and related nonsteroidal anti-inflammatory drugs (NSAIDs) led to the discovery of a host of signaling molecules derived from arachidonic acid, including prostaglandins and leukotrienes.
Drugs serve as prototypical external or environmental factors in determining how the brain adapts or maladapts over time in response to repeated perturbations. Many adaptations that occur in response to repeated drug exposure are models for adaptations to other external exposures, including stress and experience.
The ability of a drug to produce an effect on an organism is dependent on many of its properties, from its absorption to its stability to its elimination. To briefly summarize these processes, the first factor to be considered is the route of administration, which can determine how rapidly a drug reaches its target organ and which organs it affects. Oral administration typically results in a relatively slow onset of action. Parenteral describes all other routes of administration, including subcutaneous (under the skin), intraperitoneal (into the peritoneal–abdominal cavity), intravenous (into the venous system), intracerebroventricular (into the cerebral ventricular system), intrathecal (into spinal fluid), and intracerebral (into the brain parenchyma) delivery. The bioavailability of a drug determines how much of the drug that is administered actually reaches its target. Bioavailability can be influenced by absorption of the drug from the gut if administered orally. It also can be affected by binding of the drug to plasma proteins, which makes the drug unavailable to bind to its target. Moreover, it can be influenced by a drug’s ability to penetrate the blood–brain barrier if the drug acts on the brain (Chapter 2), or its ability to permeate cell membranes if the drug acts on intracellular proteins.
Drug action also depends on the stability of the drug once it is absorbed, that is, how rapidly it is metabolized to inactive congeners or eliminated from the body through urine, bile, or exhaled air. Some drugs (prodrugs) must be converted into active metabolites before they can exert their biologic effects.
Each of these factors, which can be categorized as pharmacokinetic considerations, is a critical determinant of drug action and influences both the clinical use of drugs and the process of developing new agents. However, these pharmacokinetic properties are not discussed in detail in this book because they are not, strictly speaking, related to the underlying mechanisms of drug action—the pharmacodynamic features that are the primary concern of these chapters. As an introduction to this topic, a brief description of the process by which a drug interacts with its initial protein target follows. Pharmacogenetics, which describes the influence of an individual’s genes in determining the response to a given drug, is also critical, but still in the earliest phases of understanding (see below).
Neuropharmacology is changing rapidly in response to the molecular revolution. In previous decades, neuropharmacology focused on the synapse and, more particularly, on the effects of drugs on neurotransmitters or neurotransmitter receptors. The action of drugs on synaptic targets remains an important field of investigation. The initial target of a drug generally determines the particular cells and neural circuits on which the drug acts and at the same time the potential efficacy and side effects of the pharmacologic agent. However, the molecular revolution has made it clear that the initial binding of a drug to its target—for example, the binding of a drug to a neurotransmitter receptor—is only the beginning of a signaling cascade that affects the behavior of cells and ultimately complex circuits.
When a drug binds to a protein, it affects the functioning of that protein, thereby establishing a form of allosteric regulation. A drug can conceivably bind to any site on a protein. A simple site may involve just a few contiguous amino acid residues in a protein’s primary structure, while a relatively complex site may involve discontinuous residues from the protein’s primary structure that are brought near each other by the protein’s secondary and tertiary structures. Ultimately, the three-dimensional shape, or conformation, of a binding site and the electrostatic charges distributed across the site must complement the shape and charge of the drug. The interaction of a drug with its binding site can influence the intrinsic activity of the target protein, for example, the catalytic activity of an enzyme or the conductance of an ion channel, or it can influence the ability of the protein to interact with some other molecule, such as the ability of a receptor to bind to its neurotransmitter.
In classic studies of drug mechanisms of action, a mechanism is defined by a drug’s ability to bind to an unknown receptor in tissue homogenates or on tissue sections. In these studies the drug, termed the ligand, is radiolabeled and incubated with a tissue preparation, which is washed extensively to remove loosely bound drug. A radioactive atom must be added to the drug without altering its ligand binding properties, a process that can be exceedingly difficult. The resulting ligand binding should be specific; that is, the ligand must bind to its specific target protein, which must be distinguished from binding to other proteins or even to the wall of a plastic test tube. In many cases, binding is stereoselective, or specific for only one stereoisomer of a drug. Binding also should be saturable. A limited amount of ligand binding occurs in the preparation because the amount of the specific target is limited. (A tissue preparation contains a finite amount of an individual receptor protein compared with a test tube wall, which is theoretically infinite.) Additionally, binding should attain a steady state. Time, temperature, and other conditions of incubation should enable the ligand binding to achieve a state of equilibrium.
The extent to which a ligand binds to a tissue preparation is a function of the concentration of the ligand 1–1. The total binding comprises two components: (1) specific binding, which is saturable, and (2) nonspecific binding, which is not saturable. In the ideal situation, in which binding to a specific receptor site is competitive and fully reversible in the steady state, specific binding can be defined as the fraction of total binding that can be displaced by incubating the radiolabeled ligand–tissue mixture with a large excess of unlabeled ligand. Conversely, the nondisplaceable radioactive portion of the preparation is considered nonspecific binding.
1–1
Radioligand binding assay. In this theoretical representation the amount of radioligand bound to a tissue preparation (eg, homogenate, brain slice) is a function of the concentration of the radioligand. Total binding is the total amount of binding observed. Nonspecific binding represents the nonsaturable portion of binding that is presumably not associated with the specific binding site under investigation; it is often calculated as the binding of radioligand that persists in the presence of a large excess of nonradiolabeled ligand. Specific binding is calculated as the difference between total and nonspecific binding and reflects the amount of radioligand bound to the specific binding site.

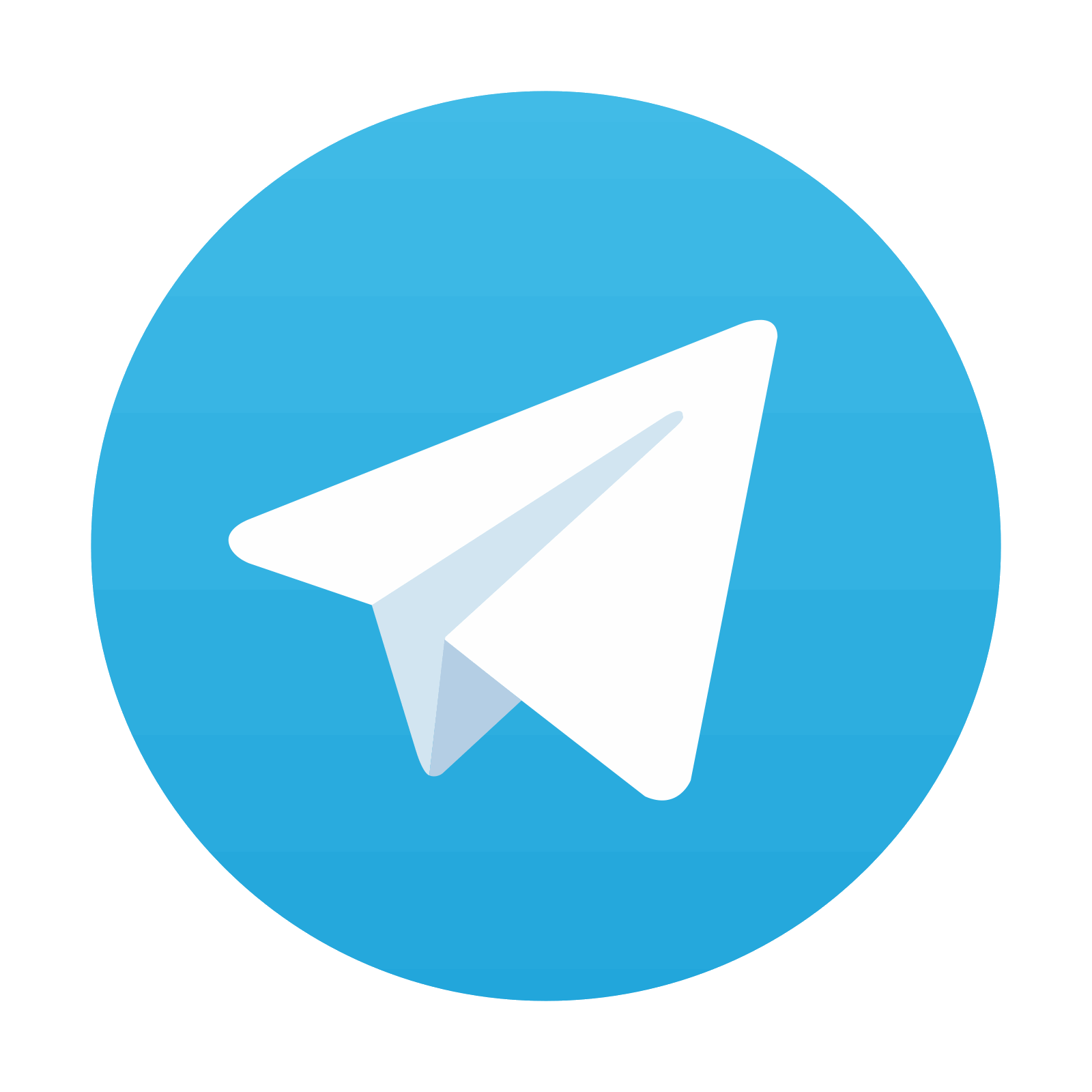
Stay updated, free articles. Join our Telegram channel
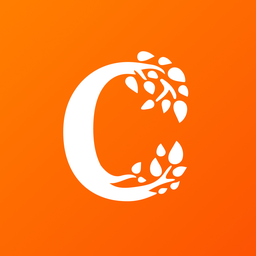
Full access? Get Clinical Tree
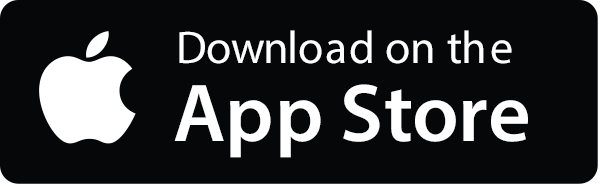
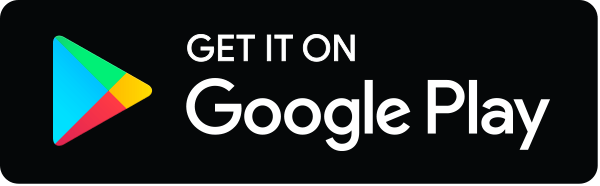
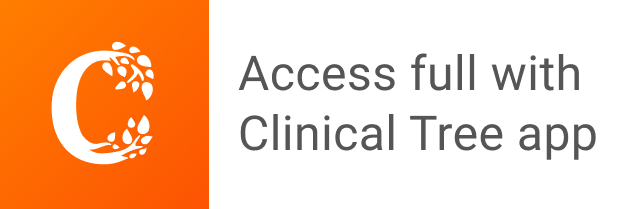