Behavioral Neurochemistry and Pharmacology
Alan C. Swann
▪ INTRODUCTION
Brain function is simultaneously organized across three spheres—spatial, neurochemical, and temporal. In this chapter we will explore the chemical organization of the brain and its relationship to spatial and temporal organization. First, we will describe the basis of neural excitability and the action potential, because these phenomena underlie chemical effects on neural activity. Next, we will describe the main transmitter systems. Finally, we will discuss integration of these systems in relation to the regulation of behavior and its disorders. Basic principles of this review include the following: (a) systems of transmitters in the brain vary in their distribution and in their chemical specificity, but have common underlying characteristics; (b) beliefs once considered axiomatic repeatedly have been disproved, and there is no reason that current views are any more immune to refutation than older ones were; (c) there are many layers of regulation of neuronal function and of interactions among transmitter systems; and (d) as a result, regulation of basic aspects of behavior that are necessary to survive overlap and involve combinations of each major type of system. These areas of overlap may be most susceptible to the subtle but pervasive disturbances underlying serious neuropsychiatric disorders.
▪ THE NEUROCHEMISTRY OF ELECTRICAL ACTIVITY AND NEURAL EXCITABILITY
The Action Potential and Neuronal Excitability
Brain function is based on the ability of groups of neurons to communicate by propagation of action potentials. To understand how specific treatments influence brain function, we will first briefly review the mechanisms regulating neural activity. These mechanisms are the ultimate targets of drug action. Figure 28.1 summarizes ionic gradients across the neuronal membrane that contribute to membrane potentials, along with three voltage-dependent cation channels prominently associated with action potentials. In summary, they are as follows:
A negative charge gradient, with the inside of the cell negative compared to the outside.
A negative concentration gradient for Na+ ions, producing potential energy that can be linked to electrical activity or transport of neurotransmitters, their precursors, and metabolites across the cell membrane.
A strongly negative concentration gradient for Ca2+, positive gradient for K+, and negative gradient for Cl−. The Cl− gradient is the basis for gamma-aminobutyric acid (GABA)-mediated inhibition.
Ion channels are pores that selectively allow ions to cross the cell membrane, increasing or decreasing the electrical potential across the membrane. Voltage-gated channels are opened
by changes in the membrane potential. Ligand-gated channels are opened by the binding of transmitters to receptor sites that can be either physically part of the ion channel or coupled to it by G proteins or other second messengers. They make the more subtle changes in potentials that, in turn, can activate voltage-gated channels.
The action potential is based on the collapse of the Na+ gradient. The normal resting membrane potential in excitable cells ranges from −60 to −90 mV. Excitatory potentials (e.g., from activation of ligand-gated ion channels by transmitters) or metabolic conditions can lower and raise the membrane potential locally. These postsynaptic excitatory or inhibitory potentials (also called receptor potentials) are summed over the time that the relevant channels are open. As the membrane potential decreases, the fast-activating Na+ channel has an increasing probability of being open. Sodium ions flood through this channel and down their electrochemical gradient, temporarily reversing the direction of the membrane potential. The voltage-dependent K+ channel opens and closes more slowly than the Na+ channel. As it opens, it allows K+ ions to leave the cell down the chemical and (temporary) electrical gradient, repolarizing the cell. The action potential is propagated along the cell surface, except where it is insulated by myelin, which speeds the spread of the action potential along axons. Between action potentials the gradients are restored by diffusion and Na+,K+-ATPase.
Energy Metabolism and Neuronal Activity: Relationship to Neuroimaging
Functional neuroimaging largely measures, indirectly, changes in the activity of Na+,K+-ATPase, which is triggered by high intracellular Na+ and acts to restore the resting ionic gradients. This process is the main source of activity-dependent energy utilization in both neurons and glia. Established methods of functional neuroimaging include positron emission tomography (PET), single photon emission computed tomography (SPECT), and functional magnetic resonance imaging (fMRI).
PET scanning with deoxyglucose measures increased glucose uptake to meet the metabolic demand of Na+,K+-ATPase activity. The brain normally relies on glucose as its energy source.
Local blood flow is generally coupled to chemical conditions associated with metabolic activity, leading to increased blood flow to electrically active areas. This is measured by PET scanning with labeled water, with SPECT, or fMRI, which uses endogenous oxygenated hemoglobin as a contrast agent.
Any condition that alters relationships between energy metabolism and electrical activity or between energy metabolism and blood flow can lead to spurious effects.
KEY POINTS
1. Neurons have a negative resting membrane potential with large gradients of Na+, K+, Ca2+ and Cl−.
2. The electrochemical Na+ gradient provides the potential energy to drive many transmembrane transport systems.
3. Receptor potentials open ion channels that increase (hyperpolarize; inhibitory) or decrease (depolarize; excitatory) the resting membrane potential.
4. If the resting membrane potential reaches a critical value, voltage-dependent Na+ channels open rapidly and allow Na+ to enter the cell, resulting in an action potential.
5. Restoration of cation gradients by Na+,K+-ATPase is the largest component of activity-dependent neuronal energy metabolism and is measured indirectly by functional neuroimaging.
▪ PROPERTIES OF MAJOR TRANSMITTER SYSTEMS
General
This section describes basic dimensions of neurotransmitter economy. Each is a potential target for pathophysiology or drug action.
Spatial Organization
Transmitter systems range from specific pathways to diffuse distribution throughout the brain. The monoamine systems are characterized by well-defined clusters of neurons in the midbrain, pons, and medulla that integrate environmental and internal stimuli to project throughout the brain. These projections can be wide projections from a compact source, as in norepinephrine, or more specifically defined, as with dopamine. Other transmitter systems, such as amino acids, are more diffusely organized.
Transmitter Economy
The function of neurotransmitter systems is affected by the synthesis, storage, release, and breakdown of the transmitters. Synthesis, in turn, is potentially sensitive to availability of precursors and regulation of synthetic enzymes. These interactions are summarized in Figure 28.2. Processes in transmitter economy have varying degrees of specificity relative to other areas of cell function. Monoaminergic systems, including catecholamines and indoleamines, have a relatively high degree of specificity, generally resulting in production of stable and useless metabolites that must be excreted, and which provide indices of transmitter utilization. Other systems, especially amino acids, are more integrated into cell metabolic pathways. Acetylcholine is an example of a monoaminergic system that is integrated into cell metabolism, with both precursors and products being integrally related to energy and phospholipid metabolism.
Regulation of Receptor Function
The availability and function of receptors is regulated by exposure to their ligands, as well as by conditions in the cell. These will be discussed with specific receptors, and include the following:
Negative feedback regulation increased exposure to ligand results in reduction of the density or function of receptors in the cell membrane.
Autoreceptors can carry out negative or positive feedback regulation.
Genetic regulation of receptors can be complex, including the presence of several protein subunits, potentially regulated by different genes, and processes such as gene splicing, RNA editing, and posttranslational processing.
Receptors can form oligomers of the same or different receptors in the membrane, changing receptor function or membrane localization.
Use-Dependent Modulation
Negative feedback control characterizes physiological systems in which recognition of a signal is crucial. Examples are regulation of monoamine receptor density and sensitivity by exposure to ligand, regulation of firing rate by autoreceptors in response to synaptic availability of
transmitter, and inhibition of firing by excessive stimulation of a system with a complementary behavioral role.
transmitter, and inhibition of firing by excessive stimulation of a system with a complementary behavioral role.
Positive feedback control characterizes systems that have to increase capacity to meet demand. Skeletal muscle is a good example. Think how bizarre life would be if muscle mass and function were regulated by negative feedback control. In excitatory amino acid systems, increased electrical activity can result in increased synaptic capacity. These mechanisms are central to learning and memory. A cellular example is posttetanic potentiation, a neurophysiological example is kindling, and sensitization of motor responses to stressors or stimulants is a behavioral example. These mechanisms all require the function of excitatory amino acid systems, increased cell calcium, and modification of presynaptic function by diffusion transmission.
Properties of Transmitter Receptors
The two main structural and functional receptor categories are G protein-coupled (metabotropic) receptors and ligand-gated ion channels. These receptors are coupled by GTPase-dependent proteins (G proteins) to second messenger, or effector, systems. Examples are alpha- and beta-noradrenergic receptors; D1, D2, D3, D4, and D5 dopaminergic receptors; most serotonin receptor types; M1 and M3 muscarinic receptors; metabotropic glutamate receptors; GABA-B receptors; most peptide receptors; purinergic adenosine and P2Y receptors; and endocannabinoid receptors.
With ligand-gated ion channels (ionotrophic receptors), the binding of agonist directly alters the function of an ion channel. Examples are muscarinic M2 receptors, GABA-A and GABA-C receptors, N-methyl-D-aspartate (NMDA) and alpha-amino-3-hydroxy-5-methyl-4-isoxazolepropionate (AMPA) glutamate receptors, and purinergic P2X receptors.
Drug Interactions with Receptors
Agonists bind to the receptor and have the same effect as its endogenous ligands. A partial agonist binds to the receptor and does the same thing as the endogenous ligand, but less effectively. The intrinsic activity of a partial agonist is the percentage of optimal effect when the receptor is fully occupied by the drug. A partial agonist with low intrinsic activity can stabilize the function of a system, because full occupation of the receptor with the partial agonist has less activity than with the endogenous agonist, but at least has some preserved function, rather than loss of function, with an antagonist.
Antagonists bind to the receptor, but binding does not have the effect of the endogenous ligand. The antagonist inactivates the receptor by preventing agonist binding.
The effectiveness of agonists and antagonists depends on their concentration and affinity for the receptor compared to those of the endogenous ligand. PET scanning with labeled receptor ligands can measure receptor effects of a drug on receptor occupancy in vivo, showing how effectively a drug competes with an endogenous transmitter.
Functions of Transmitter Receptors
Hundreds of receptor types in the brain bind scores of transmitters, activating or inhibiting dozens of effector systems. Some compounds rapidly activate or inhibit cells (generally amino acids), whereas others have slower effects that provide the background for more rapid signaling. Effects of receptor binding, whether inotropic or metabotropic, include the following:
Activating ion channels, which alter the membrane potential and therefore the probability of an action potential. Stimuli that increase the membrane potential (hyperpolarizing) are inhibitory, and stimuli that reduce the membrane potential (depolarizing) are excitatory.
Activating or inhibiting second messenger systems, which orchestrate processes relative to excitability, metabolic activity, or synthesis or storage of transmitters.
Altering transmitter release through Ca2+ channels or effects on transmitter economy.
Regulating receptor function or membrane availability, for the same (autoreceptor) or a different (heteroceptor) transmitter.
Binding to nuclear or genomic receptors or causing release of substances that bind to nuclear receptors or that interact directly with the genome.
Interactions Among Transmitter Systems
No system in the brain works alone. Interactions are described later in detail. Heterosynaptic autoreceptors alter the activity or release of other transmitters. Transmitter systems can stimulate or inhibit cells releasing other transmitters. Heterodimers or other oligomers can form with different transmitters.
KEY POINTS
1. Interacting systems of transmitters can directly influence neuronal excitability through rapid depolarization or hyperpolarization or can influence the ionic and metabolic background of neural activity.
2. Transmitter systems may be dispersed throughout the brain, may be limited to discrete areas, or may consist of systems in which discrete collections of cells project to large areas of the brain by long fiber tracts.
3. Precursors are taken up, usually by Na+-dependent mechanisms, and converted to the transmitter, which is released into the cytoplasm or stored in vesicles. Once released, the compound may diffuse away or may be taken back up into the presynaptic cell and inactivated.
4. The effectors bind to receptors that generally fall into two classes—inotropic receptors linked to ion channels and metabotropic receptors linked via coupling proteins to second messenger systems.
5. Receptor density and function are generally regulated by negative feedback; systems of functional capacity are regulated by positive feedback mechanisms that govern neural plasticity.
Monoamines
The monoamine transmitters consist of the catecholamines (dopamine, norepinephrine, and epinephrine), the indoleamines (serotonin and melatonin), histamine, and acetylcholine. These compounds share Na+-dependent uptake of precursors, synthesis that is inhibited by high product concentrations and increased with high electrical activity, Na+-dependent vesicular storage, Ca2+-dependent release from vesicular and cytoplasmic pools, multiple receptor subtypes with both excitatory and inhibitory properties, and a modulatory role in synaptic function. The pharmacology of these processes is summarized in Table 28.1. Norepinephrine, dopamine, and serotonin share additional features and have been referred to as the trimonoamine modulatory system. They are formed from essential amino acids, have stable inactive metabolites, and exercise relatively slow modulatory effects superimposed on fast transmission mediated by amino acid transmitters.
Catecholamines
This description of catecholamine system regulation is based on the diagram in Figure 28.2 and information summarized briefly in Table 28.1.
TABLE 28.1 REGULATION OF MONOAMINE AND CHOLINERGIC NEUROTRANSMITTER SYSTEMS | ||||||||||||||||||||||||||||||||||||||||||||||||||
---|---|---|---|---|---|---|---|---|---|---|---|---|---|---|---|---|---|---|---|---|---|---|---|---|---|---|---|---|---|---|---|---|---|---|---|---|---|---|---|---|---|---|---|---|---|---|---|---|---|---|
|
Transmitter Economy:
Synthesis: Epinephrine, norepinephrine, and dopamine are synthesized from the amino acid L-tyrosine. Uptake of L-tyrosine into neurons is sodium dependent, requiring energy from the extracellular-intracellular sodium gradient. The rate-limiting enzyme tyrosine hydroxylase is regulated by product inhibition (negative feedback). Increased enzyme synthesis is induced by prolonged increases in neuronal activity. The product, L–DOPA, is a common precursor of all catecholamines, but exogenous L-DOPA increases synthesis of dopamine more than norepinephrine. Dopamine beta-hydroxylase (DBH) converts dopamine to norepinephrine. Norepinephrine is converted to epinephrine by phenylethylamine N-methyltransferase.
Storage and Release: Catecholamines are stored in vesicles that also contain adenosine triphosphate (ATP) and (in the case of norepinephrine) DBH and released in a calcium-dependent process when cells are depolarized. Norepinephrine and dopamine also have a more immediately available cytoplasmic pool. Reserpine-like drugs deplete vesicular catecholamines. Stimulants increase catecholamine release; cocaine acts primarily on vesicular pools, and amphetamines act more on cytoplasmic pools. Cytoplasmic catecholamines that are not released are quickly metabolized (see later discussion).
Reuptake: Once released into the synaptic cleft, catecholamines can be taken back up into the presynaptic cell and inactivated. Inhibition of catecholamine reuptake increases synaptic availability of catecholamines and is a common mechanism in antidepressants (tricyclic, serotoninreuptake inhibitors, serotonin-norepinephrine reuptake inhibitors) and antiparkinsonian drugs (dopamine reuptake inhibitors).
Inactivation: Catecholamines are oxidized to useless metabolites that diffuse into cerebrospinal fluid (CSF) and plasma and are eventually excreted in the urine so they can be measured by
biological psychiatrists. Catecholamine breakdown starts with monoamine oxidase (MAO), a mitochondrial enzyme. There are two common forms of MAO—MAO-A (inhibited by clorgyline) and MAO-B (inhibited by deprenyl). Norepinephrine is metabolized preferentially by MAO-A, eventually forming 3-methoxy-4-hydroxyphenylglycol (MHPG), while dopamine is metabolized equally by MAO-A and MAO-B, eventually forming homovanillic acid (HVA). Transmitter that is not taken up into the presynaptic cell is inactivated initially by catechol O-methyltransferase (COMT).
biological psychiatrists. Catecholamine breakdown starts with monoamine oxidase (MAO), a mitochondrial enzyme. There are two common forms of MAO—MAO-A (inhibited by clorgyline) and MAO-B (inhibited by deprenyl). Norepinephrine is metabolized preferentially by MAO-A, eventually forming 3-methoxy-4-hydroxyphenylglycol (MHPG), while dopamine is metabolized equally by MAO-A and MAO-B, eventually forming homovanillic acid (HVA). Transmitter that is not taken up into the presynaptic cell is inactivated initially by catechol O-methyltransferase (COMT).
Clinical Correlates: The more active the system is, the more effect an exogenously administered precursor (e.g., L-tyrosine or L-DOPA) can have on transmitter synthesis. Inhibition of MAOe is a mechanism of action for a class of antidepressive agents (most of which are nonselective between types A and B), but their clinical usefulness is limited by interactions with other drugs and dietary amines. Measurement of catecholamine metabolite production can measure transmitter utilization and turnover. MHPG and HVA cross the blood-brain barrier. They can be measured in plasma, are excreted in the urine, and provide a measure of utilization of the parent amine in the central nervous system (CNS). Levels of these metabolites do not always reflect functional activity because direct inactivation of reuptake or degradative enzymes can result in increased transmitter availability in the face of decreased metabolite concentrations. Further, MHPG and HVA in peripheral body fluids is not all from the brain, because approximately half of MHPG and a greater proportion of HVA are formed peripherally. MHPG and HVA (blood, urine, or CSF) are elevated in mania.
Roles in Behavior and Brain Function: Norepinephrine has an important role in vigilance, attention, and responses to novelty. Stimulation of alpha-1 receptors (alpha-1A, B, and C) activates the protein kinase C-phospholipase C second messenger system and generally has a net stimulating effect on the postsynaptic cell. Beta-receptors (beta-1, 2, and 3) activate the adenylate cyclase system and usually have an inhibitory effect on the postsynaptic cell. In addition to neuronal effects, norepinephrine has prominent effects on cerebral blood flow via cerebral microvessels.
Clinical Effects of Norepinephrine: Stimulation of norepinephrine release results in subjective activation and anxiety in normal humans, panic or severe anxiety in individuals with anxiety or depressive disorders, and risk for hypomania in individuals with bipolar disorder. Norepinephrine is believed to be involved in anxiety, mania, and psychotic states. Drugs that enhance norepinephrine availability, including MAO inhibitors (MAOIs) and norepinephrine reuptake blockers, can be effective antidepressive agents, although direct norepinephrine receptor agonists or precursors do not seem to have this effect.
Dopamine acts through discrete systems, in contrast to the more diffuse effects of norepinephrine. Dopamine is substantially more abundant in the brain than is norepinephrine. D1 receptors are generally excitatory and stimulate adenylate cyclase; D2 receptors are more often inhibitory and inhibit adenylate cyclase. Many behavioral effects of dopamine require stimulation of both D1 and D2 receptors. D5 receptors resemble D1; D3 and D4 receptors resemble D2. Receptor blockade results in protection against overstimulation, improvement in delusions, and organization of speech and (presumably) thought, impairment of motivation, and Parkinsonian motor disorders.
Epinephrine is substantially less abundant than norepinephrine and dopamine in the CNS. It inhibits firing of the locus coeruleus.
Spatial Organization of Catecholamine Systems: Norepinephrine in the CNS has two major sources. The larger and more widespread consists of projections from the locus coeruleus, involved in regulation of attention and stimulus orientation. The locus coeruleus projects
diffusely from the pons to the cerebral cortex, hippocampus, limbic system, spinal cord, and cerebellum. The locus coeruleus is activated by novel or noxious stimuli. Locus coeruleus firing is inhibited by mu opioid receptors, epinephrine, noradrenergic alpha-2 receptors, and GABA-A receptors. The locus coeruleus is activated by cholinergic and excitatory amino acid receptors. A second noradrenergic system projects from the lateral tegmentum to the hypothalamus, amygdala, and septum and to many of the projection areas of the locus coeruleus and is involved in neuroendocrine effects of norepinephrine.
diffusely from the pons to the cerebral cortex, hippocampus, limbic system, spinal cord, and cerebellum. The locus coeruleus is activated by novel or noxious stimuli. Locus coeruleus firing is inhibited by mu opioid receptors, epinephrine, noradrenergic alpha-2 receptors, and GABA-A receptors. The locus coeruleus is activated by cholinergic and excitatory amino acid receptors. A second noradrenergic system projects from the lateral tegmentum to the hypothalamus, amygdala, and septum and to many of the projection areas of the locus coeruleus and is involved in neuroendocrine effects of norepinephrine.
Dopamine acts through several more discrete systems. These differ in terms of their regulation by autoreceptors and in terms of peptides co-released with dopamine, and include the following:
The nigrostriatal system, projecting from the substantia nigra to the corpus striatum, with prominent autoreceptor regulation, involved in the accessory motor system.
The mesocortical system projecting from the ventral tegmental area to the prefrontal, cingulate, and entorhinal cortices, without prominent autoreceptors, and involved in the initiation of action. This system has the highest firing rate and is most sensitive to precursor.
The mesolimbic system projecting from the ventral tegmental area to limbic structures such as the nucleus accumbens and amygdala, without prominent autoreceptors, involved in anticipating and responding to rewards.
The tuberoinfundibular system projecting from arcuate and paraventricular hypothalamus to the intermediate lobe of the pituitary and to the median eminence, inhibiting prolactin release.
Other medium-length systems involved in endocrine regulation, including the incertohypothalamic system and the medullary system, involving the tractus solitarius.
Short-fiber systems, including retinal amacrine cells and periglomerular cells in olfactory bulb.
Epinephrine neurons are primarily in the lateral tegmentum and medulla; they inhibit firing of the locus coeruleus and project to the spinal cord and paraventricular nucleus of the vagus nerve.
Regulation and Plasticity of Catecholamine Systems: Postsynaptic receptors are regulated by several layers of negative feedback control. Excessive exposure to agonist down-regulates receptor number and uncouples receptors from cellular effectors. Similarly, denervation or drugs that block receptors result in increased numbers of receptors in the membrane.
Noradrenergic systems are regulated by inhibitory alpha-2 autoreceptors on nerve endings or cell bodies. Blockade of norepinephrine reuptake or breakdown results in reduced noradrenergic firing rate as a result of activation of alpha-2 receptors by synaptic norepinephrine.
Dopaminergic D2 autoreceptors have a prominent role in the nigrostriatal system but not in mesocortical, mesolimbic, or tuberoinfundibular systems. Systems lacking autoreceptors are less likely to develop tolerance in response to increased dopaminergic activity.
Inhibitory postsynaptic alpha-2 and D2 receptors are activated at higher neurotransmitter or agonist concentrations. These heterosynaptic receptors regulate other transmitter systems (see later discussion of Figure 28.3). For example, heterosynaptic alpha-2 receptors can reduce firing of dopaminergic, serotonergic, and cholinergic neurons (see later discussion).
Sensitization: Dopaminergic and noradrenergic systems are susceptible to sensitization by exposure to stressors and pharmacological stimulation. Optimal sensitization appears to require activation of D2 dopamine receptors and alpha-1 noradrenergic receptors. Sensitization may be related to development of substance-dependence and recurrence in affective disorders.
![]() ▪ FIGURE 28.3 Interactions among monoaminergic systems and glutamate. Diagram of the main inhibitory (−) and excitatory (+) links among norepinephrine, serotonin, dopamine, and glutamate systems. |
KEY POINTS
1. Catecholamines are synthesized from L-tyrosine. The rate-limiting step is production of L-DOPA by tyrosine hydroxylase. DBH catalyzes formation of norepinephrine from dopamine.
2. Catecholamines are stored in vesicles and can be released from either vesicles or cytoplasm.
3. Catecholamines are taken up by Na+-dependent carriers and metabolized by MAO or COMT.
4. Firing rates of catecholaminergic neurons are under feedback control by autoreceptors.
5. Norepinephrine projects diffusely and is involved in alertness, orientation, and novelty or stress.
6. Dopamine has several relatively discrete systems and is involved in motor activity, motivation, and reward systems.
Serotonin
Synthesis: L-Tryptophan is the amino acid precursor of serotonin. L-Tryptophan is converted to 5-hydroxytryptophan, by tryptophan hydroxylase, the rate-limiting enzyme of serotonin synthesis. With increased activity, administration of precursor becomes more effective in increasing serotonin synthesis. Tryptophan hydroxylase is sensitive to product inhibition and is induced by prolonged activation.
Storage and Release: Serotonin is stored in vesicles and released in a Ca2+-dependent manner resembling that of catecholamines. Newly synthesized cytoplasmic serotonin is preferentially
released and, if not released, is promptly metabolized. Vesicular serotonin, like catecholamines, can be depleted by reserpine and cocaine.
released and, if not released, is promptly metabolized. Vesicular serotonin, like catecholamines, can be depleted by reserpine and cocaine.
Reuptake: Serotonin, like catecholamines, is removed from synapses by a sodium-dependent reuptake system. Inhibition of serotonin reuptake increases synaptic availability of serotonin and is a common mechanism of antidepressive drugs. Reuptake blockade results in stimulation of autoreceptors and a resulting compensatory reduction in firing rate.
Inactivation: As with catecholamines, the most important enzyme in serotonin inactivation is MAO, especially type A. The main metabolite of serotonin is 5-hydroxyindoleacetic acid (5-HIAA), which can be measured in CSF, plasma, and urine.
Clinical Correlates: The more active that serotonergic neurons are, the more effectively administration of precursor (L-tryptophan) can increase serotonin synthesis. Most 5-HIAA in peripheral body fluids is from peripheral sources (primarily the gastrointestinal tract) rather than brain. 5-HIAA in CSF is low in patients with aggressive or impulsive behavior and with violent or severe suicidal behavior.
Roles in Behavior and Brain Function: Serotonin has prominent roles in behavior and in motor regulation. In the regulation of goal-directed, drive-related behavior, it appears complementary to dopamine, acting to inhibit many drive-related and consummatory behaviors, to inhibit aggressive behavior, and to reduce impulsive behavior. Destruction of serotonergic systems increases impulsive behavior in animals, but only if the dopaminergic system is intact. Serotonin interacts with dopamine in the accessory motor system and is important in regulating sleep and neuroendocrine function.
Spatial Organization: The midbrain raphe nuclei project widely to the cerebral cortex, limbic system, hypothalamus, basal ganglia, and spinal cord.
KEY POINTS
1. Serotonin regulation is largely analogous to that of catecholamines.
2. Serotonin is synthesized from its precursor, L-tryptophan, by tryptophan hydroxylase. Serotonin is released from cytoplasmic or vesicular pools, is retrieved from the synapse by an Na+-dependent reuptake system, and is inactivated in the cell by MAO-A.
3. Serotonin has prominent behavioral roles that often are complementary to those of dopamine, reducing consummatory or drive-related behavior, aggression, and impulsivity. Low serotonin function is associated with impulsivity, aggression, and suicide risk.
Acetylcholine
Synthesis: Acetylcholine is synthesized from acetyl coenzyme A and choline, catalyzed by choline acetyltransferase. Both precursors have prominent metabolic roles other than acetylcholine synthesis. Acetyl coenzyme A comes mostly from glycolysis, followed by oxidation of pyruvate or from the citric acid cycle. Choline is derived from phospholipids or can enter cells through Na+-dependent uptake. Availability of choline can limit the rate of acetylcholine synthesis when the cell is active. About half of the choline required for acetylcholine synthesis is recycled after synaptic hydrolysis of acetylcholine.
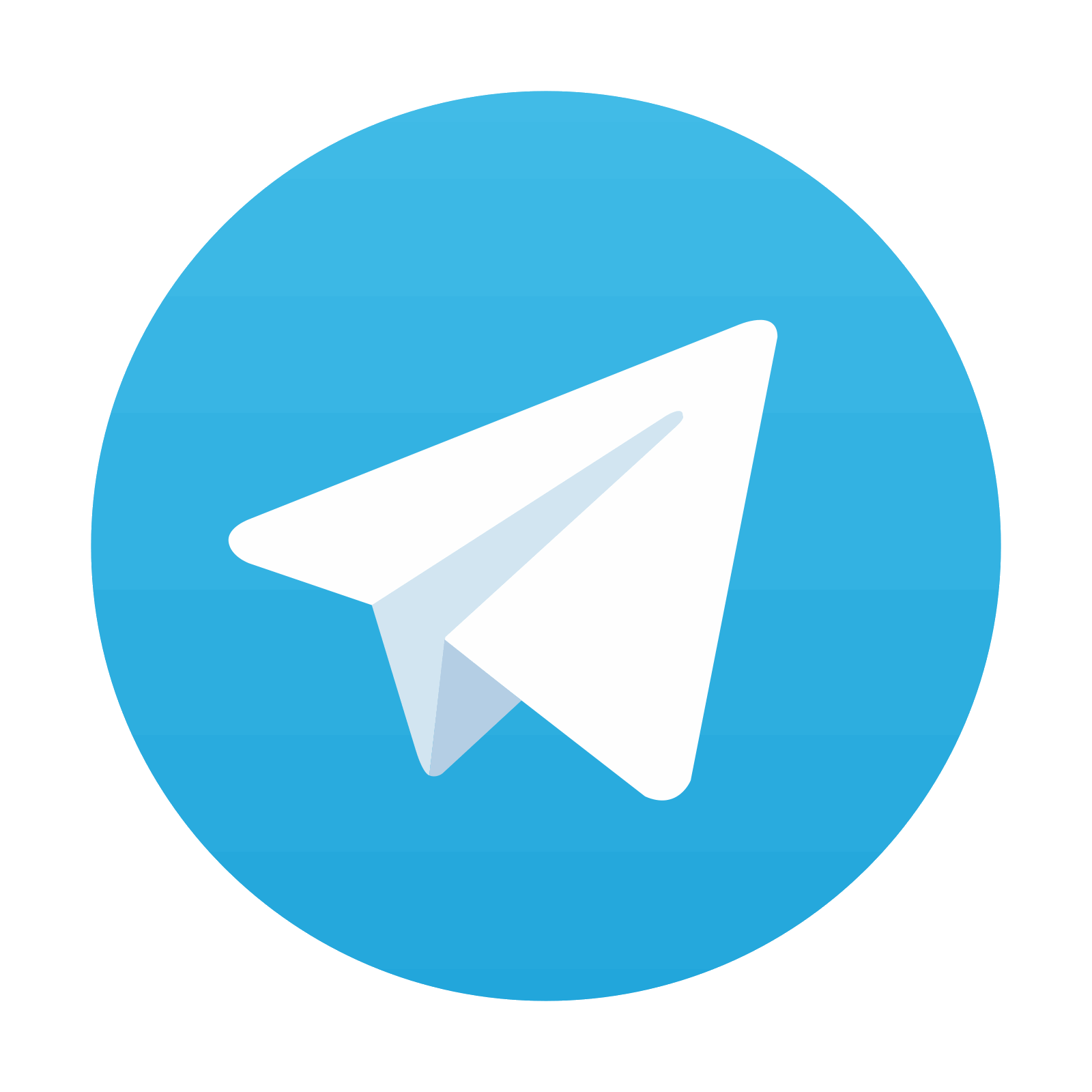
Stay updated, free articles. Join our Telegram channel
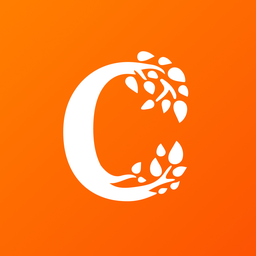
Full access? Get Clinical Tree
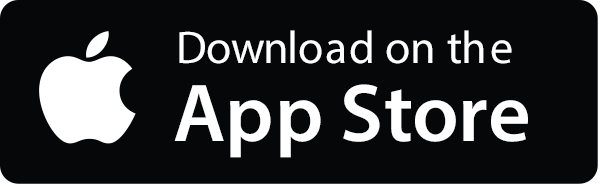
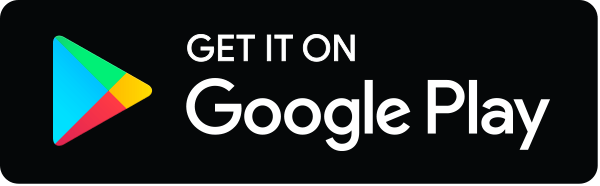
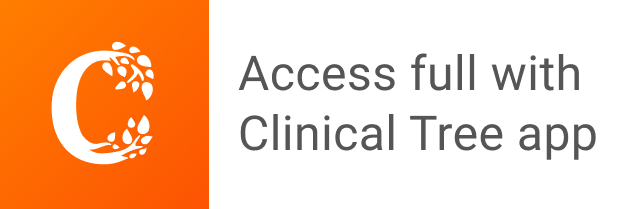