30 Biologics for Spinal Fusion Wellington K. Hsu and Jeffrey C. Wang Spinal arthrodesis presents several obstacles to even the most experienced spine surgeon. Despite the use of internal fixation, pseudarthrosis still occurs in 10 to 15% of all spine fusion surgeries.1–4 The significant rates of pseudarthroses and reports of operative morbidity from the harvest of autograft can limit the success rates of primary spine fusion in certain patients. In addition, the stringent biologic environment created from pseudarthrosis formation presents a complicated array of problems and unpredictable outcomes after further surgical intervention. Dense fibrous tissue, intervertebral disc, and muscle cells commonly encountered during revision procedures have been found to inhibit host bone repair.5 Because the success rates of fusion in this poor osteoinductive environment are relatively low, recent studies have been directed toward the development of new bone graft substitutes to improve outcomes in both primary and revision procedures. The arena of spinal biologics has expanded to include autogenous bone graft, allograft, autologous cells, demineralized bone matrix (DBM), recombinant growth factors, and tissue engineering therapies. Continued research has been conducted to identify the ideal bone graft substitutes for spine surgery, which should be osteogenic, biocompatible, user-friendly, and cost-effective, and should provide structural support.6 In this chapter, we review the preclinical and clinical data regarding the use of biologics in the induction of spine fusion. Autogenous cancellous bone graft remains the gold standard material for the treatment of bone loss challenges because it alone offers three of the necessary components for bone repair: osteoinductive signals from associated growth factors, osteogenic cells, and an osteoconductive matrix. Several animal models have provided insight into the incorporation of cancellous grafts into host bone.7 Histologic and biomechanical evidence indicates that autogenous cancellous graft offers incorporation into host bone in 6 to 12 months. Two distinct phases have been described in the incorporative process: an early phase characterized by active bone resorption and formation, and a late phase identified by creeping substitution.8 By 1 year after surgery, complete remodeling and incorporation of the graft are seen.8 Both autogenous cortical and cancellous bone grafts are currently used in spine fusion procedures. The majority of autografts harvested contain cancellous bone. Although without compressive strength, cancellous grafts have a large trabecular area, which encourages the consolidation of the fusion mass. The bone graft ultimately becomes denser than surrounding host bone.9 Cortical grafts offer structural support for the spinal column and are used for a wide range of spinal defects (0.5 to 25 cm). This type of graft is incorporated slower with less efficiency than cancellous allograft. Furthermore, cortical grafts undergo resorption before host incorporation. Stress fractures have also been reported in longer grafts (12 to 25 cm).10 The use of autologous bone graft in the setting of spine fusion typically presents several concerns for the surgeon. The elderly patient population presents unique clinical problems such as osteoporosis, poor bone stock, and anesthetic risks of increased operative time. Studies have shown that the harvest of autogenous graft leads to clinically significant perioperative and postoperative morbidity.11–14 Finally, the options for biologic supplementation in the setting of revision spine surgery are decreased when previous iliac crest bone graft was harvested. Allograft bone offers advantages over autogenous bone graft because there is an abundant supply of graft material and the morbidity associated with autograft harvest is avoided. By decreasing antigenicity and thus, the host immune response, the process of freeze-drying avoids a localized inflammatory response and possible rejection of the material. As a result, this leads to increased graft incorporation after implantation.15,16 On the other hand, this modification also decreases osteogenic activity and hinders host vascular invasion. When compared with autografts, the loss of osteoinductive capabilities leads to a higher incidence of nonunion and delayed union.7 The risk of disease transmission from musculoskeletal tissue donors exists from the use of allografts; however, this has been reported to be low.17 Cancellous allograft bone chips and cortical allografts have been used extensively in spinal fusions. Anterior femoral ring allografts have been shown to successfully induce spinal fusion (range 52% to 98%) when posterior instrumentation is used.18–20 Clinical evidence has suggested that the use of tricortical allograft may offer comparable fusion rates and clinical outcomes to iliac crest autograft after revision anterior arthrodesis of the lumbar spine.21 However, studies comparing outcomes between allograft and autograft in the posterolateral arthrodesis of the lumbar spine are more controversial. An et al22 reported a prospective comparison of autograft and allograft preparations in the posterolateral arthrodesis of the lumbar spine and concluded that allograft was less efficacious in achieving radiographically evident spine fusion (80% versus 50% successful fusion, respectively).22 Another prospective study concluded that ethylene oxide-treated allograft mixed with autogenous bone graft was significantly less reliable in inducing spinal fusion compared with iliac crest autograft alone.23 In studies involving the cervical spine, single-level anterior fusions comparing allograft and autograft bone have revealed higher fusion rates with the use of iliac crest autograft (78% versus 92%, respectively).24–26 However, in these same studies, satisfactory fusion rates using allograft bone were reported (78 to 90% fusion). Evidence involving multilevel cervical fusion or corpectomies show a much higher fusion rate with the use of autograft versus allograft (83% versus 47%, respectively).4,22,26,27 DBM is created through the acid extraction of the mineralized phase of bone. The preparation of DBM was originally characterized by Urist et al28,29 and then modified by Reddi and Huggins.30 Methods of processing follow the same initial steps; however, additives and refining techniques are different depending on the source and company involved. Commercial preparations also use different carriers such as glycerol, hyaluronic acid, gelatin, and calcium sulfate powder. This has led to wide biologic variability in terms of osteoinductive potential in vivo.17,31,32 DBMs have been found to have rich osteoconductive capabilities but questionable osteoinductive ability. DBMs have been shown to induce rapid revascularization, serving as an excellent osteoconductive scaffold.33 However, osteoinductive capacity of DBM is dependent not only on the original donor, but also on the different commercial sterilization and handling methods. Sterilization by ethylene oxide and use of gamma irradiation, for example, have been found to significantly reduce osteoinductivity.34,35 Commercial preparations of DBMs also use different carriers that may impart unpredictable biologic effects. For example, a large number of DBMs have been combined with glycerol to help convert the allograft to a putty form. This carrier has been demonstrated to be lethally toxic to athymic rats in a dose-dependent manner.36 Other studies have confirmed the renal toxicity of glycerol in large doses37; however, despite these findings, in more than 10 years of use of these DBMs in humans, there have been no reported cases of renal toxicity related to this carrier. Despite their wide use and variable processing methods, DBMs have been tested in few animal models and laboratory studies. Recent studies have demonstrated the variability of these preparations in inducing osteogenic activity in an intramuscular animal model,38 a rat femoral defect,39 and a rat spine fusion model.17 In a study using a rat spine fusion model, widely variable osteoinductive potential was demonstrated using different commercially available DBM preparations.17 Histologic analysis of the spines 8 weeks after DBM implantation demonstrated variable rates of fusion, amount of new bone formation, and presence of residual DBM. This wide variability of biologic activity of DBMs is likely influenced by the associated donor the carrier, and the assorted demineralization and sterilization methods used.17 DBMs have been used successfully as bone graft extenders to promote spinal fusion and the healing of long bone nonunions.6,40–42 Certain formulations have also induced successful spine arthrodesis when used alone or in conjunction with autograft, bone marrow, or ceramic carriers.37,43–47 Additional studies have combined the use of autologous bone marrow and DBM in healing osseous defects.48,49 There is a need for further study of the influence of donor age and sex, processing, and success of DBMs.50 Both the historic lack of Food and Drug Adminstration (FDA) oversight in the United States and the wide variety of donors to supply the graft contribute to the variability in outcome from the use of DBM. Furthermore, different preparations are combined with different carriers that impart variable osteoconductive and osteoinductive capabilities. However, it is important to note that DBMs should be used clinically solely as bone graft extenders and not substitutes. Because all DBMs do not have the same biologic potential, the optimal DBM for each clinical situation needs to be determined. Bone marrow contains osteoprogenitor cells and growth factors that actively recruit host mesenchymal stem cells (MSCs) to undergo osteoblastic differentiation. Recent research has reported the ability of bone marrow to stimulate bone formation.51 Autologous cells provide significant osteoinductive capabilities through osteogenic cells, however, when used alone, they lack localized structural support. For this reason, the combination of bone graft substitutes and autologous marrow has been assessed in tibial nonunions, bone cysts, and comminuted fractures associated with bone loss.45,49,52,53 MSCs in autogenous bone marrow are capable of developing into mature osteoblasts when exposed to the appropriate growth factors.54–58 Furthermore, culture expansion of MSCs has been shown to amplify the number of osteoprogenitor cells in vitro.58,59 In a preclinical critical-sized defect model in canines, culture-expanded MSCs exhibited superior healing rates when compared with bone marrow.58 These results show promise for the use of MSC therapy in spinal fusion, particularly in the elderly patient population. Bone marrow cells are readily accessible through aspiration from the posterior iliac wing, and a recent study has recommended the harvest of smaller volumes (2 mL) of bone marrow from any one location to obtain a higher concentration of osteoblast progenitor cells.59 Muschler et al reported the efficacy of concentrating bone marrow-derived cells from bone marrow aspirates using a selective cell attachment technique in a canine posterior segmental spine fusion model.60 Their results suggest that when used with a bone marrow clot, an enriched cellular composite graft of concentrated bone marrow cells induced a greater spine fusion mass volume in vivo than cancellous bone matrix alone.60 Together, the use of autologous cells with a carrier offers components for bone repair akin to that of autogenous bone graft. Complications are subsequently avoided, such as those associated with bone graft harvesting and the low risk of infection. However, there are concerns about the potential variability in human bone marrow cellularity as well as an age-related decline in progenitor cells.61 Although the benefits are supported by a strong theoretical basis and success in animal models, further clinical studies into using autologous cells as a bone graft substitute in spine fusion are needed. The discovery of bone morphogenetic proteins (BMPs) by Urist in 196562 has led to a diverse area of research dedicated to the identification and characterization of osteoinductive growth factors. Members of the transforming growth factor-B (TGF-β) superfamily, BMPs have been proposed for several applications in orthopaedic surgery.63 Although 14 different BMPs have been reported,64 much of the recent study in the literature has focused on BMP-2, 6, 7, 9, and 14 (MP-52). Recombinant BMP-2 (rhBMP-2) and BMP-7 (or osteogenic protein-1, rhOP-1) have been evaluated in numerous preclinical models, and successful healing in long bone defects has been reported.63,65–67 Similar findings have been demonstrated in spinal arthrodesis models in animals.68–71 In the United States, FDA approval has been granted for the use of rhBMP-2 to enhance anterior spinal fusion72 and rhOP-1 to supplement posterior spine fusions for pseudarthrosis (under a humanitarian device exemption).73 Recombinant BMP-2 has been shown to reproducibly heal the lumbar spine in rodents and nonhuman primates.65,71,74–82 Furthermore, rhOP-1 has also demonstrated consistent bone healing properties in rodent and sheep models.76,83–86 Results from these studies suggest that the use of rhBMP results in similar if not superior fusion rates with biomechanically stronger fusion masses when compared with autogenous bone graft.65,71,74–82 Vaccaro and colleagues recently demonstrated the efficacy of rhOP-1 putty (3.5 mg rhOP-1 with 1 g type I collagen) in the enhancement of posterolateral lumbar arthrodesis.87 In a randomized, prospective, multicenter study, 36 patients with degenerative spondylolisthesis were treated with either rhOP-1 or autogenous iliac crest bone graft in an uninstrumented posterolateral fusion following a decompressive laminectomy. At the one-year time point, 74% (14 of 19 patients) of the rhOP-1 and 60% (6 of 10 patients) in the autograft groups achieved a successful clinical and radiographic posterolateral arthrodesis using static and dynamic plain radiographs, which was not statistically significant.87 These authors concluded that fusion in the absence of internal fixation with the use of rhOP-1 putty was safe and yielded comparable results to that of iliac crest bone graft. Similarly, Boden et al reported the successful clinical use of rhBMP-2 in the healing of a posterolateral spine fusion both with and without instrumentation in a comparison study involving 25 patients.88 Clinical improvement as defined by the mean Oswestry Disability Index score (6 weeks postoperatively) was greatest in the rhBMP-2 treatment only group. Interestingly, the authors concluded that the use of a higher dose of recombinant growth factor in nonhuman primates (1.5 to 2.0 mg/mL) than in rodents (0.2 to 0.4 mg/mL), was required in healing a posterolateral spine fusion.89 To date, it remains unclear why concentrations of BMP a million times greater than that found in the human body are required to successfully induce a spinal arthrodesis.90–92 Follow-up studies utilizing rhBMP-2 have confirmed its successful use in inducing a posterolateral spinal fusion diagnosed by computed tomography (CT).93,94 Glassman et al reported the use of a large INFUSE kit (12 mg rhBMP-2/ACS, Infuse Bone Graft, Medtronic Sofamor Danek) in the posterolateral fusion bed as equivalent fusion success to iliac crest bone graft (ICBG).94 The authors concluded that INFUSE can effectively substitute for ICBG for both one- and two-level posterolateral instrumented fusions. Dimar and colleagues published 2-year radiographic results from an FDA interventional device exemption study comparing ICBG and rhBMP-2 combined with a compression-resistant matrix (CRM) carrier for single posterolateral fusions. The authors demonstrated that patients in the BMP/CRM group experienced significantly higher fusion rates yet had less surgical time and blood loss than the ICBG group (88% versus 73%; P = 0.051).93 The importance of associated carriers with BMP was elucidated when Barnes et al95 reported the results of rhBMP-2 delivered on an absorbable collagen sponge wrapped around a bulking agent consisting of biphasic calcium phosphate and collagen in a posterolateral fusion model in rhesus monkeys. Results from this and other studies suggest that the required dosage of rhBMP-2 for spinal arthrodesis can be reduced by optimizing the delivery of growth factor by combining the strengths of different carriers.96 On the other hand, carriers such as fibrin glue have been shown to inhibit bone formation induced from rhBMP and may provide protection from heterotopic ossification and diffusion of protein to undesirable adjacent areas.97 Because the treatment of spinal pseudarthrosis is fraught with relatively poor outcomes and potential complications, the interest in the utilization of rhBMP for these clinical challenges is on the rise. With the use of different preclinical pseudarthrosis models, recombinant growth factors may eventually prove to be a more appropriate bone graft option than other existing choices including ICBG. With the use of a nicotine-exposed rabbit lumbar pseudarthrosis model, OP-1 was found to increase the expression of crucial genes in bone repair such as angiogenin, vascular endothelial growth factor, and bone morphogenetic proteins.98 In fact, these authors concluded that application of a single BMP in relatively high concentrations to a biologically stringent environment can induce angiogenic and osteogenic gene expression greater than that seen with autologous graft. Despite the overwhelming evidence in support of the routine use of rhBMPs in the enhancement of spinal arthrodesis, several studies have suggested potential complications with its clinical use. Smucker et al99 reported that 27.5% of 69 patients who underwent anterior cervical spine fusions using rhBMP-2 had a clinical significant neck swelling event compared with only 3.6% of patients in the non-rhBMP2 group. Other studies have confirmed the finding that the use of rhBMP-2 in the anterior cervical spine can be problematic.100,101 Furthermore, the use of rhBMP-2 in transforaminal lumbar interbody fusion has been reported to lead to significant vertebral bone resorption in a total of 22 of 32 lumbar levels studied postoperatively with a CT scan.102 These authors concluded that rhBMP-2 was the direct cause of resorption, which led to graft subsidence and prevented solid radiographic union in a significant number of cases.102 Tissue engineering options are attractive as an adjunct to spine surgery because of their ability to closely approximate the biology of autologous bone graft. Furthermore, many of these treatment strategies offer a continuous delivery of recombinant protein, which may enhance spinal fusion rates in a compromised biologic environment. Protocols utilizing the combination of bone graft substitutes, recombinant proteins, and/or gene transfer systems could offer additional options for spine arthrodesis. Gene therapy involves the in vitro transfer of genetic material to cells to stimulate in vivo expression of a targeted protein. Gene therapy systems are composed of the DNA sequence, a vector, such as a virus, to mobilize the genetic material in question, and target cells to express the protein. Transduction of target cells can occur in several different ways. In vivo techniques involve the direct injection of the vector into the site of bony repair, whereas ex vivo systems require the harvest of responding cells and transduction with a virus in an in vitro setting. In general, ex vivo systems have been studied more extensively because of higher transduction efficiencies when compared with in vivo methods. Early results using BMP gene transfer via an in vivo technique revealed successful fusion only in immunocompromised animals.103–105 Since then, several immunocompetent animal studies have demonstrated the efficacy of gene therapy in the healing of long bone defects106–108 and spinal fusion.109,110 Wang et al reported the initial results with the use of ex vivo adenoviral gene transfer in a posterolateral spine fusion model in immunocompetent rats.71 Histomorphometric analysis revealed that coarse trabecular bone had formed from cells generated by adenoviral gene transfer whereas thin, lace-like bone formed from that of recombinant BMP.71 This finding has also been confirmed in a rat femoral critical-sized defect model.107 The success of gene therapy has also been reported in higher animal models.105,111,112 In one study, harvested mesenchymal stem cells (MSCs) from pigs were used as targets for adenoviral-mediated transfer of the BMP-2 gene.112 The transduced cells were then used to successfully heal the thoracic disc spaces of three pigs through an anterior thoracoscopic injection. Radiographic and histologic examination confirmed bridging bone in all six disc spaces treated with adenovirus; little bone formation was seen in the control injections.112 The utilization of growth factors other than BMP-2 with ex vivo gene therapy in bone formation has also been described. In addition to BMP-2 and BMP-7, recent reports have shown the successful use of other genes, such as BMP-4,113 BMP-6,114 and BMP-9.115 Furthermore, Viggeswarapu et al110 demonstrated solid posterolateral arthrodesis of the spine in a rabbit model from the adenoviral delivery of LIM mineralization protein-1 (LMP-1) into the fusion bed. LMP-1 is an intracellular protein that has been shown to function as an upstream regulator for several BMPs. Although recombinant BMP homodimers such as BMP-2 and BMP-7 have been used successfully in animal models of spinal fusion, recent studies have suggested that the coexpression of multiple BMPs can lead to the formation of heterodimeric BMPs.116–118 These studies also suggest that heterodimeric BMPs may have more potent osteoblastic potential than their homodimeric counterparts. Using a rat posterolateral spine fusion model, Zhu and colleagues119 tested the in vivo and in vitro capacity of combination gene transfer using BMP-2 and BMP-7 in comparison to single BMP gene transfer. A549 cells were cotransfected with adenoviral vectors encoding for BMP-2 and BMP-7, and with each vector alone. The authors found that the animals treated with cotransfection of both BMP genes experienced a significantly greater number of mechanically stable spinal fusions with higher bone fusion mass assessed via CT scanning when compared with single gene transfer.119 These findings suggest that the use of heterodimeric BMPs may possibly lead to lower required doses of recombinant protein and fewer responding cells in tissue engineering. Further optimization to gene therapy protocols have been directed at the use of different cellular delivery vehicles. Although multiple types of target cells have been used to deliver growth factors for bone formation, bone marrow stromal cells have been the most widely used. However, studies demonstrate that bone marrow contains a relatively low percentage of MSCs that have the capability of differentiating into osteoblasts.59 Moreover, this ratio is further diminished in elderly patients, those with metabolic diseases, and osteoporosis.61 Because of the inherent limitations of bone marrow stromal cells, several other gene delivery vehicles have been utilized. Buffy coat cells, which are a concentrate of white blood cells and platelets derived from either bone marrow or blood, have been used successfully to produce spine fusions in both rat and rabbit models after transduction with LMP-1.110 Other target cells used successfully in gene transfer strategies include periosteal cells,111 C2C12 myoblasts,120 and muscle-derived stem cells.121,122 Recent interest has been directed toward human liposuction aspirates, obtained from human fat, which contain an abundance of pluripotent progenitor cells termed processed lipoaspirate cells. These cells can undergo purification steps to produce adipose-derived stem cells (ADSCs) that have demonstrated the ability to differentiate into cells of chondrogenic, osteogenic, myogenic, and adipogenic lineage. The prospect of using processed lipoaspirate cells is attractive because they offer a significantly higher yield of mesenchymal stem cells than bone marrow,123 can be obtained through a minimally invasive procedure under local anesthesia, and are widely available among the patient population.124 Studies using adipose cells as gene delivery vehicles have reported initial success in the induction of bone formation in several different animal models.125–127 One study from our laboratory has successfully described the use of ADSCs as cellular delivery vehicles in the induction of spine fusion in a rat posterolateral spine fusion model using an adenoviral vector.126 Microcomputed tomographic and plain radiographic analysis of fusion masses revealed over twice as much bone formation in animals treated with ADSCs transduced with the adenoviral vector carrying the BMP-2 gene when compared with those from high-dose recombinant BMP-2.126 Spinal arthrodesis is complicated by challenges including osteoporotic bone, a stringent biologic environment, and poor local vascularity. Bone graft substitutes should provide for consistent arthrodesis without the morbidity associated with autogenous graft. As a whole, however, these substances vary widely in regard to available data, and careful evaluation is necessary to identify the appropriate use of various bone graft agents. In making these critical decisions, surgeons must assess the host biologic environment and must ensure that the four critical elements are present to promote bone repair: the presence of bioactive factors, responding cells, matrix, and an adequate vascular supply. Currently established treatment options including the use of allograft, autograft, or DBM, may soon be regularly supplemented with recombinant growth factors or products from tissue engineering. Although its cost effectiveness must be assessed, use of these novel biologic substitutes may be used to enhance bone repair in more stringent biologic environments. The body of evidence reporting the efficacy of recombinant BMP in clinical studies has grown considerably over the past 5 years. Since the first report of BMP-induced osteoinduction in a clinical trial,72 additional studies have reported the superiority of rhBMP-2 to the use of autogenous bone graft.72,83,89,93,128,129 Moreover, patients treated with rhBMP-2 alone have been found to show more rapid and significant clinical improvement after spine fusion.89 Furthermore, multiple investigators have demonstrated the osteoinductive versatility of recombinant BMP using multiple approaches. Recent evidence has supported the efficacy of rhBMP in posterolateral, interbody, and transpedicular approaches in inducing radiographic and histologic spine fusion.83,88,89,102,128,129 However, despite excellent clinical results, many concerns still exist for the routine use of recombinant growth factors. Clinical studies, which confirm the safety from the use of rhBMP-2 in humans,87,130 fall short in evaluating possible long-term effects. Several complications have also been associated with its use in both the cervical and lumbar spine.99,101,102 Furthermore, the cost of recombinant BMPs currently precludes its routine use in spine arthrodesis; further study will be necessary to delineate the clear indications in which BMPs should be used. Despite promising preclinical results from gene therapy, safety concerns have hindered the progress of tissue engineering in the clinical setting. Recent interest has been dedicated to characterizing the lifespan of transduced cells, duration of gene expression, and systemic effects on the host genome after the use of gene therapy. Additional studies in higher animal models are needed to assess the long-term effects of gene therapy before its clinical use in humans is widely accepted. With additional investigation into its safety profile in humans, the use of gene therapy may one day become a reality in the field of musculoskeletal surgery in the future. Multiple avenues of research exist in the development of biologic substitutes for the enhancement of spine fusion. The continued laboratory and clinical characterization of spinal biologics will ultimately offer spine surgeons multiple options in the arena of spine fusion. 14. Younger EM, Chapman MW. Morbidity at bone graft donor sites. J Orthop Trauma 1989;3(3):192–195 29. Urist MR, Silverman BF, Buring K, Dubuc FL, Rosenberg JM. The bone induction principle. Clin Orthop Relat Res 1967;53:243–283 62. Urist MR. Bone: formation by autoinduction. Science 1965; 150(698):893–899 65. Cook SD, Dalton JE, Tan EH, Whitecloud TS III, Rueger DC. In vivo evaluation of recombinant human osteogenic protein (rhOP-1) implants as a bone graft substitute for spinal fusions. Spine 1994; 19(15):1655–1663 73. Food and Drug Administration. Device approval letter to Stryker. Available at: http://www.fda.gov/cdrh/pdf2/H020008a.pdf. 96. Akamaru T, Suh D, Boden SD, Kim HS, Minamide A, Louis-Ugbo J. Simple carrier matrix modifications can enhance delivery of recombinant human bone morphogenetic protein-2 for posterolateral spine fusion. Spine 2003;28(5):429–434
Autograft
Allograft
Demineralized Bone Matrix
Autologous Cells
Bone Morphogenetic Proteins
Tissue Engineering
Discussion and Conclusion
References
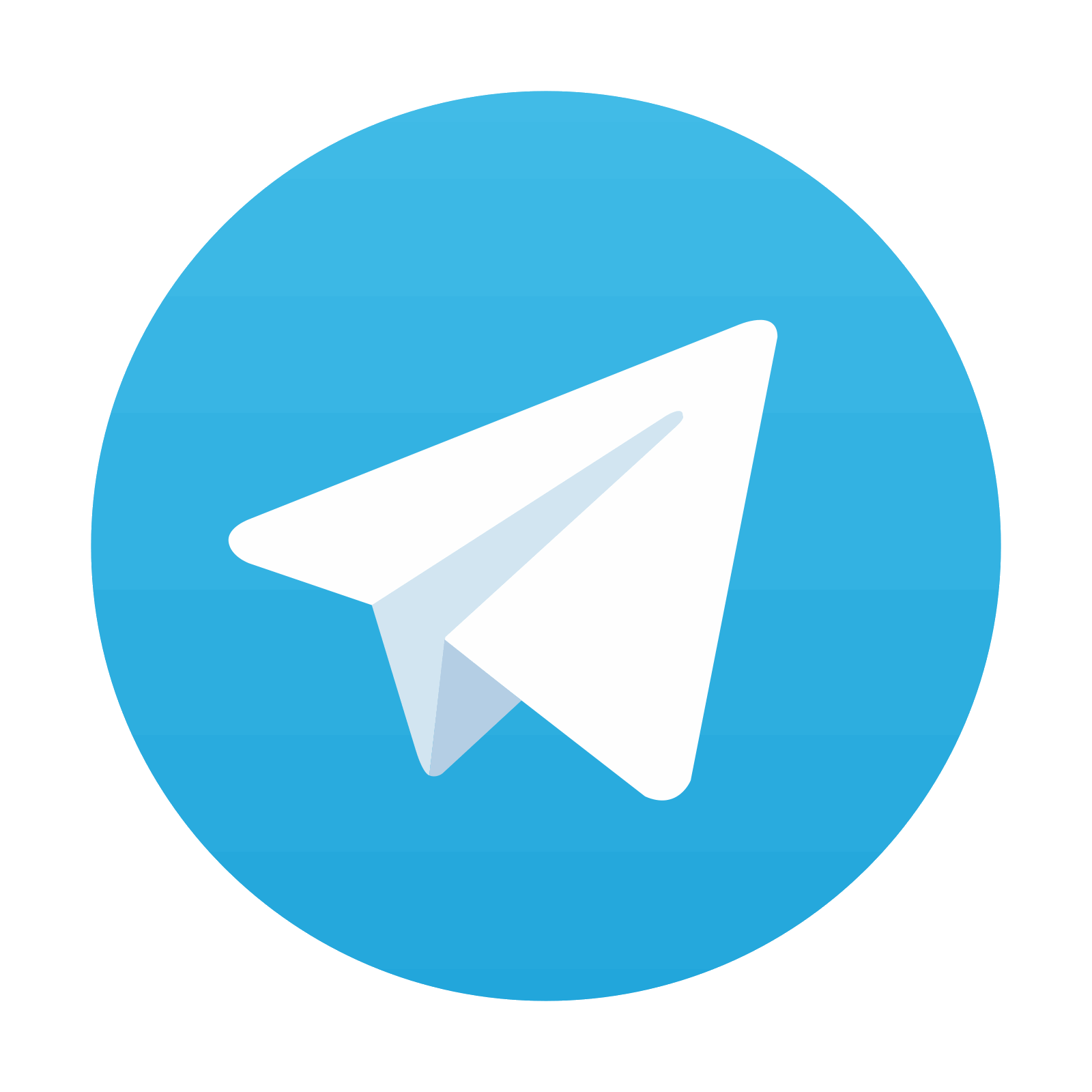
Stay updated, free articles. Join our Telegram channel
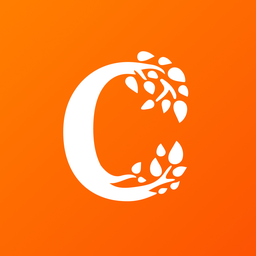
Full access? Get Clinical Tree
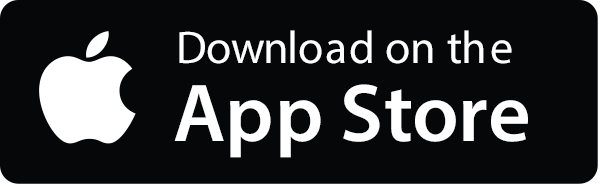
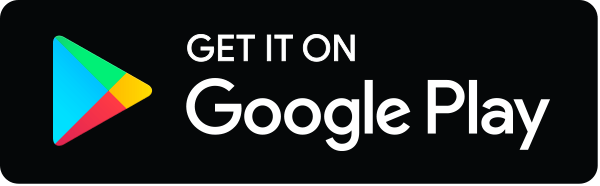