Study
Phase
Type of cells
Route of injection
Time after stroke
Sample size
Results
Kondziolka et al., Neurology 2000
I
Cultured neuronal cells differentiated from a human teratocarcinoma cell line
Intracerebral
7–55 months
12
Treatment is safe and feasible
Kondziolka et al.,J Neurosurg 2005
II
Cultured neuronal cells differentiated from a human teratocarcinoma cell line
Intracerebral
1–6 years
14 + 4 controls
Functional improvements with no change in primary outcome measure
Savitz et al., Cerebrovasc Dis 2005
I
Dissociated cells from primordial porcine striatum
Intracerebral
1.5–10 years
5
Study stopped after cortical vein occlusion and seizures
Rabinovich et al., Bull Exp Biol Med 2005
I
Frozen samples from dissociated human fetal nervous and hemopoietic tissues
Subarachnoid space
4–24 months
10
No serious complication with potential functional improvement
Suarez-Monteagudo et al., Restor Neurol Neurosci 2009
I
Autologous bone marrow mononuclear cells
Intracerebral
1–10 years
5
Safe
Barbosa da Fonseca et al., Exp Neurol 2010
I
Labeled autologous bone marrow mononuclear cells
Intra-arterial
59–82 days
6
Safe and feasible, cell homing in the brain and other internal organs
Bang et al., Ann Neurol 2005
I/II
Cultured autologous human mesenchymal stem cells
Intravenous
< 7 days
5 + 25 controls
Safe and feasible, potential functional benefit
Lee et al., Stem Cells 2010
I/II
Cultured autologous human mesenchymal stem cells
Intravenous
< 7 days
16 + 36 controls
Safe and feasible, potential functional benefit
Battistella et al., Regen Med 2011
I
Labeled autologous bone marrow mononuclear cells
Intra-arterial
< 90 days
6
Safe and feasible
Savitz et al., Ann Neurol 2011
I
Autologous bone marrow mononuclear cells
Intravenous
24–72 h
10
Safe and feasible
Honmou et al., Brain 2011
I
Autologous bone marrow mesenchymal stem cells
Intravenous
36–133 days
12
Safe and feasible
Moniche et al., Stroke 2012
I/II
Autologous bone marrow mononuclear cells
Intra-arterial
5–9 days
10 + 10 controls
Safe and feasible
Parsad et al., Indian J Med Res 2012
I
Autologous bone marrow mononuclear cells
Intravenous
7–30 days
11
Safe and feasible
Bhasin et al., Cerebrovasc Dis Extra 2011
I
Cultured autologous bone marrow mesenchymal cells
Intravenous
7–12 months
6 + 6 controls
Safe and feasible
Bhasin et al., Clin Neurol Neurosurg 2013
I/II
Autologous bone marrow mononuclear and mesenchymal cells
Intravenous
3–24 months
20 + 20 controls
Safe and improvement of modified Barthel Index
A review of experimental stroke studies reveals cell survival is still an ongoing challenge. Injection of transplanted stem/progenitor cells into the center of the stroke infarct is a reasonable choice since stem/progenitor cells potentiate endogenous repair processes that are taking place in the peri-infarct tissue. In addition, the stroke core is a potential space that can receive considerable volumes of injected cell suspension. However, the infarct core presents as an unfavorable recipient with lack of trophic support and continuous inflammation [36, 37]. As an alternative, cells can be injected into the intact tissue surrounding the infarction. However, an injection could be damaging to the very same tissue that undergoes repair. Therefore, we are yet to find a balance between safety and efficiency in cell therapy for stroke.
Researchers have explored two alternative approaches for a safe and efficacious delivery of stem/progenitor cells. Endovascular delivery of stem/progenitor cells, either intra-arterial or intravenous injection of stem/progenitor cells, has been subject of many experimental and some clinical studies. Experiments have shown the injected cells are attracted to the stroke site and promote functional recovery [35, 38]. While endovascular injection of cells presents a feasible method for multiple sessions of cell therapy, it seeds the entire body with transplanted cells. Although short-term safety for such a body-wide cell delivery has been established for few cell products [39–45], long-term safety is yet to be determined. Moreover, intravenous injection of cells leads to entrapment of cells into internal organs [46] and, in some cases, no migration to the brain lesions takes place [47]. Although a directed delivery is possible with intra-arterial injections, cell engraftment is still not ideal [38]. This low yield of cell delivery to the site of stroke can explain the limited efficiency of intravascular method in promoting recovery that is observed in few experiments directly comparing intravascular cell injection with a direct intraparenchymal cell delivery [47, 48]. These limitations in intravascular delivery of cells for treating ischemic stroke highlight the importance of promoting stem/progenitor cell survival and integration in a direct cell injection to the stroke core.
In this chapter, we focus on application of biomaterials as a feasible, flexible, and effective approach to localize the cells into the infarct core, isolate cells from inflammatory attack and harmful factors in the microenvironment of ischemic brain lesions, and further promote their differentiation and integration by providing them with a proper niche.
Biopolymer Hydrogels: Chemistry and Physics
Chemical Composition
Biomaterials have been widely used as scaffolds for cells transplanted to the central nervous system (CNS). They have been synthetized from either natural or synthetic materials (Table 13.2). Natural sources include proteins such as Matrigel [18], collagen [49, 50], fibronectin [51], and fibrin [52], polysaccharides such as chitosan [53], agarose [54], alginate [55] and methylcellulose [56], hyaluronan (HA) [14, 57] and acellular tissue matrix [58]. Many of these molecules are physiologically found in the extracellular matrix (ECM), and therefore their use has the advantage of promoting cell signaling through their binding sites for mammalian cells. In addition, chitosan and agarose have available functional groups that facilitate chemical alterations [59]. From a translational perspective, natural molecules are clinically used as dermal fillers, lubricants, wound sealants, and surgical sponges [60] which facilitate their approval for use from safety and regulatory standpoint.
Table 13.2
Biomaterials commonly used in therapies for the brain and spinal cord lesions
Natural | Synthetic |
---|---|
Collagen | Poly-lactic-co-glycolic acid (PLGA) |
Fibrin | Poly (acrylonitrile)/poly(vinyl chloride) or PAN/PVC |
Fibronectin | Oligo (ethylene glycol) fumarate (OPF) |
Matrigel | Poly(N-isopropylacrylamide) or PNiPAAM |
Chitosan | Polyethylene glycols (PEGs) |
Agarose | |
Alginate | |
Methylcellulose | |
Hyaluronan | |
Acellular tissue matrix |
On the other hand, by assembling a list of design criteria for certain scaffold applications, one can produce synthetic polymers with defined compositions, polymerization and degradation rates, and mechanochemical properties. Synthetic molecules are produced in consistent ways that reduce data variability. Moreover, by being biologically inert, synthetic molecules decrease interaction with inflammatory cells in the brain and improve implant biocompatibility. Poly-lactic-co-glycolic acid or PLGA [61, 62], poly (acrylonitrile)/poly(vinyl chloride) or PAN/PVC [61, 62], oligo (ethylene glycol) fumarate or OPF [65], poly(N-isopropylacrylamide) or PNiPAAM, and polyethylene glycols (PEGs) [66] are common examples of synthetic molecules used as scaffolds in the CNS. It is noteworthy that polyesters such as PLGA have been used as drug delivery vehicles, orthopedic fixation devices, and absorbable sutures [67, 68], and they have established a history of clinical safety which accelerates their approval in clinical trials.
It is important to know that limited chemical and biophysical interactions of the aforementioned molecules in the context of biological complexity of NSPCs and poststroke brain tissue often necessitate a combination of natural molecules, synthetic polymers, or a conglomerate of both to compose the optimized scaffold product for the best cell survival and integration following transplantation in stroke.
Physical Structure
Microspheres
Biological scaffolds can be further categorized based on their micro- and nanostructure. The earliest attempt to use scaffolds in order to improve cell viability goes back to the 1990s when scientists noticed adrenal chromaffin cells demonstrate a poor cell survival and efficacy upon transplantation to the experimental lesions of Parkinson’s disease (PD). To improve the outcome of cell therapy, they injected cells attached to collagen-coated dextran or glass beads as microcarriers [69, 70]. This form of “particulated” matrix promoted cell survival and corrected for dopamine deficits in the striatum. Another particulated matrix, gelatin beads, produced under the name of Spheramine®, was shown to promote survival and function of human retinal pigmented epithelium cells in an experimental model of PD [71]. Alginate beads were able to protect encapsulated cells against Huntington’s disease where survival of transplanted rat choroid plexus cells was improved following quinolinic acid (QA) injection [72]. Most relevant to our review, a scaffold of PLGA microspheres promoted integration of NSPCs following injection into the stroke brain cavity [36]. These studies demonstrated the importance of a viable three-dimensional (3D) matrix in transplanting cells to the brain lesions.
Besides scaffolding for cell transplantation, microspheres have been widely used to bypass the blood–brain barrier and deliver drugs, proteins, peptides, viral constructs, DNA, small interfering RNA (siRNA), and other therapeutics into the CNS. Based on their chemical properties, microspheres have a degradation rate that determines releasing pace of the compound encompassed within microparticles. This property ensures a reliable sustained release of an encapsulated molecule. Having multiple microspheres with different degradation rates gives a powerful tool to sequentially release growth factors and optimize tissue repair. For instance, to maximize proliferation of NSPCs as well as neuroprotection following stroke, it may be important to deliver sequential factors, such as epidermal growth factor (EGF) followed by release of the cytokine erythropoietin (EPO). The Shoichet group has achieved this goal by coating EPO-containing nanoparticles with a layer of poly(sebacic acid) that retards the release of EPO for about 7 days [56] and therefore optimizes tissue repair following stroke.
The slow-release capability of microspheres can be combined with cell attachment on their outer surface (Fig. 13.). In fact, microspheres could be sintered at low temperature to form a stable 3D microenvironment for cell transplantation [15]. Survival of transplanted cells attached on a 3D matrix of microspheres could be further augmented when microspheres gradually release survival and differentiation factors and thereby exert synergistic effects of adhesion and growth factor signals on cells. This strategy has been employed in treating PD where glial-derived neurotrophic factor (GDNF)-loaded PLGA microspheres promoted the efficacy of embryonic ventral mesencephalon dopaminergic cells attached on their exterior surface [73].
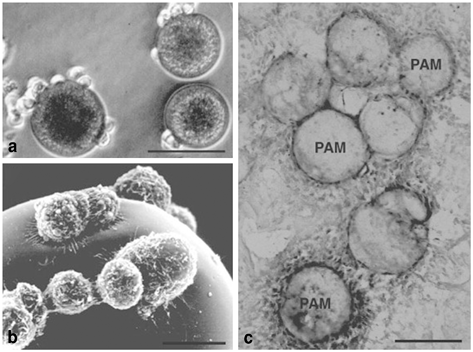
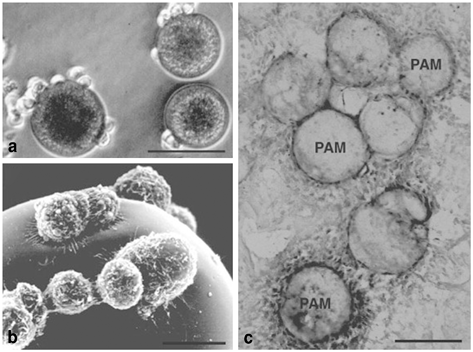
Fig. 13.1
Pheochromocytoma (PC)12 cells attach on pharmacologically active microcarriers (PAM) that release nerve growth factor (NGF). Optical (a) and surface electron (b) microscopy has documented adherence of PC12 cells on the PAM external surface. Two weeks after transplantation into the brain NGF-releasing PAM are photographed and stained for tyrosine hydroxylase (TH) positive cells (c). Scale bars a, c: 50 µm; b: 5 µm. (Adapted with permission from Tatard et al., Biomaterials 2005 and Delcroix et al., Biomaterials 2010)
Hydrogel Biopolymers
Hydrogels with their unique properties are suitable candidates for tissue engineering and promoting cell therapy. They are hydrophilic polymer networks made of long chains of monomers that are cross-linked and form a stable network. With their strong propensity for water, they absorb over ninefold of their dry weight. This makes hydrogels a highly porous and hydrated network (Fig. 13.2) that allows free diffusion of oxygen and nutrients and therefore support survival of encapsulated cells. It is noteworthy that the ECM of the brain consists of HA-based porous hydrogel network that lends support to neuronal, glial, and vascular elements of the brain and allows migration of different cell types, including NSPCs, in health and disease [74‒76]. It is therefore compatible with the brain structure to use hydrogels as vehicles for cell delivery. The mechanics of their polymer network renders hydrogels as viscoelastic materials [77]. Their elastic property ensures they retain their shape after polymerization, and hence hydrogels will stay conformed to the boundaries of lesion following polymerization inside lesion cavity. The viscous component of their mechanics resists their flow out of lesion cavity, and, as a result, hydrogel will remain in place.
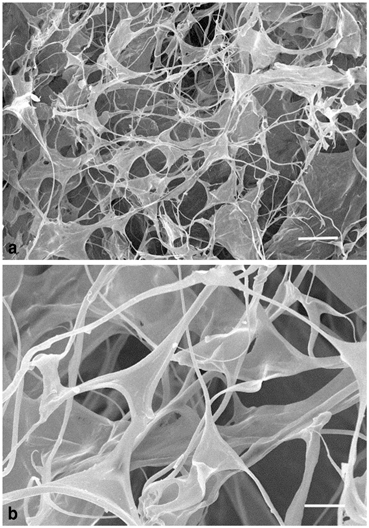
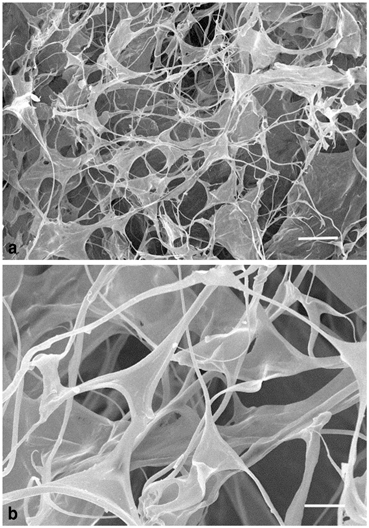
Fig. 13.2
A hyaluronan hydrogel shown at lower (a) or higher (b) magnification using scanning electron microscope. Scale bars a: 50 µm; b: 20 µm. (Reproduced with permission from Hou et al., Neuroscience 2006)
Polymerization
Gelation, or polymerization, defines the process of changing from a liquid to solid state and producing a gel from a pre-gel solution. It includes formation of covalent cross-linking bonds between long polymer chains. This process generally involves reactive functional groups on the backbone chain such as acrylates, thiols, or polyesters. In contrast to chemical cross-linking, some polymers such as agarose, Matrigel, alginate, and collagen undergo physical cross-linking by formation of hydrogen bounds [61]. The degree of cross-linking will predict important parameters of hydrogels, such as mechanical properties, porosity, degradation rate, and functionality of hydrogels [61]. These parameters contribute to the function and efficacy of hydrogels in promoting survival and differentiation of encapsulated cells, as well as integration into the host brain tissue.
Following addition of cross-linkers, hydrogels reach their maximum strength after polymerization time has elapsed. The polymerization speed is controlled by the affinity of cross-linker to backbone functional groups, density of cross-linking points, and concentration of cross-linkers. Therefore, an optimum gelation time provides a unique opportunity to access the stroke core with a minimally invasive method—insertion of a fine needle—and injection of the hydrogel before gelation occurs. Gelation can also be initiated by environmental changes in temperature [78, 79] or pH [78‒80], which provide further tools to provoke gelation following changes in temperature or pH of the lesion microenvironment. In situ gelation will allow hydrogels to conform to the boundaries of an irregularly bordered lesion and establish a proper contact with peri-infarct tissue [14], which is necessary for implant integration.
Stiffness and Stability
Hydrogels can be synthetized in a variety of stiffnesses, but those aimed for application in the CNS need to match the brain in elasticity (see “Biopolymer Hydrogels: Promoting Integration”). Stiffer gels are generally associated with higher levels of cross-linking. Therefore, a degree of cross-linking is desired to produce gels with a compressive elastic modulus between 300–450 Pa that match the brain in its mechanical properties [81]. The extent of polymer cross-attachments also defines degree of polymer stability. Loosely attached polymers will lead to swelling. Swelling of implanted hydrogel, however, is not significant in vivo since the swelling gel is confined by counterforces of the surrounding brain tissue that resists increases in overall size [82].
Porosity
The density of cross-linking points and length of cross-linking arms determine gel porosity or pore size, a crucial contributing factor to hydrogel function. The encapsulated cells need exchanging nutrients, oxygen, and waste with surrounding tissue by diffusion across borders of hydrogel; this exchange is mainly determined by hydrogel pore size. The ability of cells to migrate and axons to extend processes inside the gel also depends on size and connectivity of hydrogel pores. It is possible to guide formation of new connections by growing axons within a hydrogel with an interconnected network of pores [83]. In the case of slow-releasing hydrogels, the release rate is also predicted by mesh size, as well as size and polarity of molecules. In addition, ingrowth of blood vessels is integral to formation of any tissue larger than the diffusion limits of the extant vasculature. In stroke, the vasculature is distorted by the infarct, tissue adsorption, and vascular network remodeling. It would be desirable to induce neovascularization of a transplant so that it can achieve adequate blood supply.
Degradation
Degradation is another important parameter in application of biopolymer matrices, and it leads to dissolution and disappearance of hydrogels. It is the result of breakage of cross-linking or labile bonds within polymers through hydrolytic or enzymatic cleavage [84‒86]. Degradation has important functional implications for cell scaffolds as hydrogels can be synthetized to degrade with different speeds: A slow rate of degradation leads to gel digestion only after encapsulated NSPCs have developed their own matrix and are therefore ready to integrate into the host brain tissue. In the case of a quick gel digestion, early removal of a foreign object will attenuate inflammation and subsequent foreign body response. Therefore, optimizing degradation rate according to the pace of matrix deposition by encapsulated NSPCs will maximize tissue repair after stroke. In designing biopolymers, it is also important to know the degradation products to make sure they will be cleared away and are nontoxic leading to damage and inflammation. PLGA polymer, the most commonly used degradable synthetic polymers [66], is particularly safe from this aspect since the final degradation products are nontoxic molecules, CO2 and H2O [87]. This fact has contributed to the biocompatibility of PLGA constructs as carriers of NSPCs in the brain after stroke [36].
In the next two parts, we focus on interaction of hydrogel with encapsulated stem/progenitor cells to promote their survival and differentiation, and that of hydrogel with peri-infarct tissue to facilitate integration of transplanted biopolymer matrix to the surrounding normal brain tissue.
Biopolymer Hydrogels: Impact on Cell Survival and Differentiation
As discussed in “stem cell Therapy for Stroke,” stem/progenitor cells are promising therapeutic options for stroke. However, poor survival of transplanted cells limits their efficacy. Transplanting stem/progenitor cells within a hydrogel matrix resembling their physiological niche improves cell survival through several mechanisms (Fig. 13.3) discussed below. Although the repair capabilities of stem/progenitor cells are not merely exercised through neural differentiation and integration to the host neural circuitry, using transplanted matrix to promote neural differentiation of stem/progenitor cells can further augment their repair potential. In addition, encapsulating multipotent stem/progenitor cells in a finely crafted biopolymer matrix may ensure cells will not take aberrant differentiation paths to produce tumors such as teratomas.
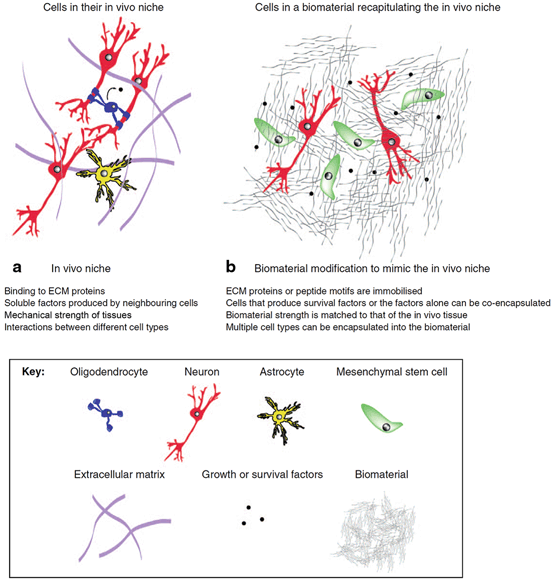
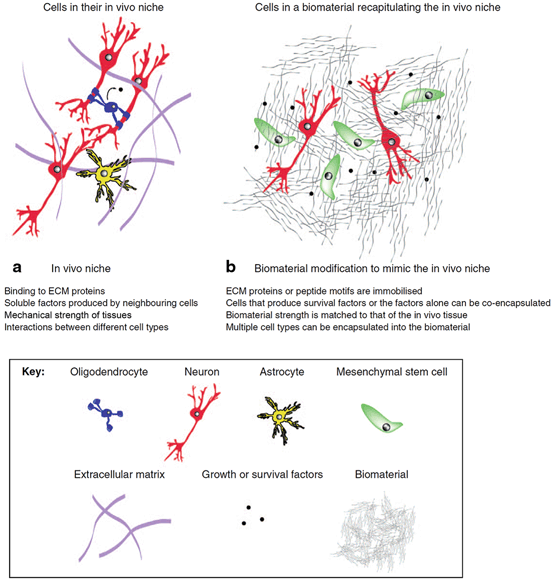
Fig. 13.3
Biomaterials provide a permissive microenvironment for transplanted cells by resembling the in vivo niche. a A combination of extracellular matrix (ECM) chemical and mechanical characteristics, soluble factors, and cell–cell interactions compose the in vivo niche that contribute to physiological functions of cells. b Biomaterial solutions for cell therapy in stroke mimic various mechanical, biochemical, and cellular components of in vivo cellular niche. (Reproduced with permission from [12])
Promoting Transplanted Cell Survival
There is a multitude of factors contributing to low cell yield following transplantation to the stroke infarct core. We will show that biomaterial scaffolds improve cell survival by targeting mechanisms of cells death. An immediate example of technical difficulties that lead to poor transplant survival is seen in the injection of cells as a thin suspension of cells injected into the brain. The tissue retains some but inevitably restricts part of the injected volume and part of cell suspension flows back to the pial surface and may diffuse into subarachnoid space. Mixing cells with biopolymer hydrogels that have viscosity equal or above the infarcted tissue improves retainability and targets entire cell population to the focus of stroke [14, 88]. This not only improves number of transplanted cells, and therefore promotes recovery, but also avoids distribution of stem/progenitor cells across the CNS, a safety concern in stem/progenitor cell clinical trials.
Adhesion
Besides hematologic cells, the majority of human cells are adherent to their underlying matrix. This adhesion plays a crucial role in keeping cells alive and functioning. In the early 1990s, scientists described cell apoptosis due to absence of cell anchorage, and they coined the term anoikis [89]. In cell therapies, detached cells are kept and injected within an aqueous medium. Upon transplantation into the infarct core, cells’ attachment to the ECM is complicated due to ischemia damage and digestion by influx of inflammatory cells. In fact, anoikis has been shown to contribute to cell death in other models of CNS injuries [90]. Cell encapsulated in a particulated or hydrogel 3D matrix is provided with a cell-adhesive environment from the moment of injection. Many natural biopolymer gels, such as collagen and fibronectin [91], and HA [88], are naturally found in the stem/progenitor cell niche matrix and therefore engage directly with receptors on stem/progenitor cell. A different type of 3D matrix, fibronectin-coated PLGA microspheres, has also promoted survival of NSPCs following transplantation to the ischemic lesion [36].
Although synthetic polymers are generally nonadhesive for cells, they can be functionalized by covalently attached ECM proteins. In this approach, all the active sites of ECM proteins, which signal for survival, proliferation, migration, and differentiation, are included. Moreover, protein loss due to protein desorption is minimal [92]. However, covalent binding of proteins requires protein modifications that carries the risk of protein functional loss due to alterations in protein active sites, denaturation, or inaccessibility of active sites in a random orientation of molecules [62]. As a solution, the polypeptide sequence within active sites of proteins, which interacts with cell integrin receptors, has been identified and industrially synthetized with inclusion of an active moiety for attachment to the polymer backbone. Oligopeptide sequences from ECM protein, such as fibronectin (Arg-Gly-Asp or RGD) and laminin (Ile-Lys-Val-Ala-Val or IKVAV, and Tyr-Ile-Gly-Ser-Arg or YIGSR), have been incorporated in biomimetic solutions to produce hydrogels with the functional elements found in stem cell/progenitor niche and improve cell survival in transplant therapies.
Trophic Support and Protection from Inflammation
Stem or progenitor cells both in culture and in their in vivo niche are exposed to growth factors to promote their survival. Following transplantation into the infarct core, cells are placed in a microenvironment lacking supportive glial cells that produce survival factors. This picture becomes more complicated by presence of inflammatory cells secreting proapoptotic factors such as interleukin (IL)-1 and tumor necrosis factor (TNF)-α [93]. Biopolymer matrices promote cell survival by: (1) attenuating inflammatory response and (2) providing trophic support. Synthetic polymers generally do not interact with inflammatory cells, and they can therefore stealth encapsulated cells from inflammatory cells. Immunogenicity of transplanted stem/progenitor cells, due to interspecies genetic differences or genetic manipulation of cells, plays a major role in elimination of transplanted cells by the host immune system. Therefore, concealing cells from inflammatory cells will impede the host-versus-graft attack [94]. Some biopolymers, such as high molecular weight HA, are known to silence inflammation and protect encapsulated cells from inflammatory insult [88].
Cellular scaffolds can be further rendered prosurvival by releasing factors that suppress inflammation or induce cell survival. Simply mixing soluble molecules in hydrogel exposes cells to a transient and high concentration of molecules that could potentially exert toxic effects. A fast diffusion and degradation of soluble molecules leads to quickly diminishing concentrations of molecules that limit any potential benefits. To circumvent this problem, growth factors could be immobilized to the scaffold backbone by covalent linkage. Alternatively, a variety of sustained-release strategies could be employed. Cyclosporine A, a potent anti-inflammatory drug, has been incorporated into PLGA microspheres or HA–methylcellulose composites as drug reservoirs and slowly released over the brain cortex [95]. PLGA particles have been further used to deliver dexamethasone, another potent immunosuppressor, to the CNS [96]. The inflammatory response could be further suppressed by incorporating neutralizing molecules for reactive oxygen species (superoxide dismutase mimetic metalloporphyrin macromer or MnTPPyP-Acryl; [97]) or TNF-α antagonizing peptides [98].
Epidermal growth factor (EGF) and basic fibroblast growth factor (bFGF or FGF-2) are two molecules providing prosurvival and pro-proliferative support for transplanted NSPCs [99, 100]. The natural tendency of bFGF to attach to heparin has been utilized in heparin-modified HA gels to gradually release bFGF [101]. Incorporating bFGF in a PEG hydrogel has promoted survival of NSPCs [102] and human mesenchymal stem cells [103]. A combination of platelet-derived growth factor (PDGF) and neurotrophin (NT)-3 gradually releasing from fibrin scaffolds has induced survival of encapsulated neural progenitor cells [104]. In this example, a cell scaffold has significantly improved cell survival through synergistic effects of cell adhesives and trophic support. In another example of this synergistic effect, mesencephalon neuronal cells attached to PLGA microspheres received trophic support by sustained release of GDNF from microspheres [73].
Controlling Differentiation of Encapsulated Cells
The secretory profile of stem/progenitor cells and their differentiation, both mechanisms contributing to neural repair after stroke, are affected by their surrounding microenvironment. This includes the ECM composition of proteins, adjacent cells, humoral growth factors (such as brain-derived neurotrophic factor or BDNF, ciliary neurotrophic factor or CNTF, NT-3, PDGF, GDNF, and nerve growth factor or NGF), and biophysical properties of their neighboring matrix. These elements are present in the unique niche of neural stem or progenitor cells to cue them for self-renewal and differentiation. Hence, any successful application of biologic scaffolds for cell delivery should consider mimicking a similar combination of factors.
A seminal study by Discher’s group [105] revealed that focusing on biochemical factors as the sole regulators of stem cell differentiation is a simplistic view. They showed that the stiffness of the substrate matrix influences the differentiation of mesenchymal stem cells. More interestingly, the stiffness of tissues drives differentiation in resident populations of stem/progenitor cells by a very characteristic and specific manner, as when cultured on substrates mimicking bone, muscle, or brain in their stiffness, mesenchymal cells were inclined to make mature osteoblasts, myocytes, or neurons, respectively [105]. This indicates how tissue elasticity, besides biochemical factors, provides signals for renewal of specialized cells. Other groups have found the same concept in regulating NSPCs (Fig. 13.4). NSPCs’ proliferation and self-renewal increase on hydrogels below 10 kPa (compressive modulus) and maximize around 3.5 kPa [110]. Neural differentiation of NSPCs, however, requires lower elasticities below 1 kPa that in fact resembles the mechanical properties of the brain [81]. Hydrogel scaffolds with their flexibility in degree of cross-linking offer a very powerful tool to optimize self-renewal and differentiation. For instance, NSPCs transplanted in a 3.5-kPa matrix will mount a proliferative response and following a slow degradation of hydrogel biopolymer and descent of stiffness to < 1 kPa, their self-renewal slows and NSPCs start differentiating to neuron. Besides differentiation, other proregenerative functions of stem/progenitor cells such as their secretory profile are also controlled by matrix stiffness [111].
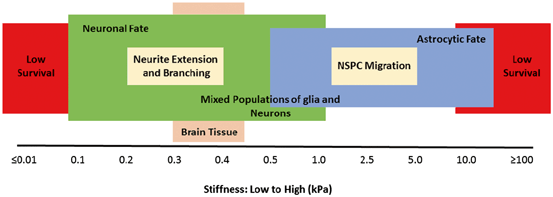
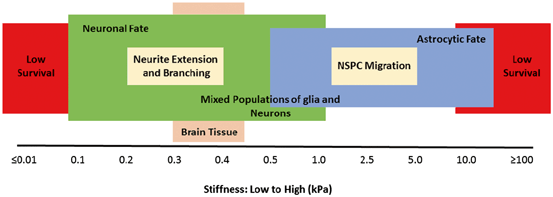
Fig. 13.4
Biopolymer stiffness determines survival, fate, and function of neural stem/progenitor cells (NSPCs). NSPCs do not survive well in a matrix with very low or very high stiffness. From those cells that survive at the lower stiffness, they differentiate more prominently towards a neuronal cell fate. In contrast, those growing at the higher stiffness tend to develop astrocytes. Neurite extension and NSPC migration also depend on biomaterial stiffness and maximize at lower and higher stiffnesses, respectively. (Original data published elsewhere [57, 81, 84, 102, 105‒109]. Reproduced with permission from [61])
The incorporation of ECM proteins, such as collagen [112, 113], laminin [114], and fibronectin [112], has been used to mimic stem/progenitor cells niche and promote their differentiation. Stem/progenitor cells start to modify their microenvironment after transplantation and secrete their own ECM. Using cross-linkers amenable to cleavage by MMPs facilitates remodeling of hydrogel scaffolds by their encapsulated stem/progenitor cells [115] and promotes their differentiation and axon extension. Differentiation could also be promoted by other biophysical factors that affect cell–substrate interactions, such as micropatterned surfaces [116], addition of electrically charged monomer methacrylate [117], and forcing into distinctive cell morphologies [118].
Biopolymer matrices have been employed to present a range of growth factors to the population of encapsulated stem/progenitor cells and drive their proliferation and differentiation. This includes strategies to immobilize growth factors or incorporate them in a slow-releasing reservoir. Agarose-immobilized PDGF or chitosan-immobilized interferon-γ has led to differentiation of NSPCs to oligodendrocytes [119] or neurons [120], respectively. Alternatively, a sustained provision of NT-3 [121] or differentiation factor dibutyryl cyclic-adenosine monophosphate [122] from PLGA microspheres have induced neural differentiation in stem/progenitor cells. Differentiation has also been augmented by hydrogel delivery of CNTF, NT-3, PDGF, GDNF, or NGF [55, 104].
In the future, encapsulating cells within biopolymer scaffold with a selected set of factors to drive differentiation may be an alternative to transplanting pre-differentiated stem/progenitor cells. Instead, a scaffold with finely crafted elasticity, surface charge, micropattern structure, and ECM proteins composition together with timely release of appropriate proliferation and growth factors would be a viable approach to direct differentiation of encapsulated stem/progenitor cells for a prolonged time after transplantation.
Biopolymer Hydrogels: Promoting Integration
The integration of transplanted stem/progenitor cells into the host tissue is critical for tissue repair and necessary for any functional improvement following cell transplantation in stroke. Integration in the context of cell therapy is defined as “the ability of the transplanted cells to interact with the host tissue in a beneficial way” [66]. It depends on the ability of transplanted cells to reach and interact with the host tissue and likewise access of host cells to the implanted matrix. In situ gelation of a biopolymer matrix maximizes the contact between scaffold and the host tissue and consequently improves cell movement from and to the scaffold. Optimized porosity, chemistry, and elasticity, and inclusion of ECM proteins are important factors in scaffold design that promote cell migration and lead to assimilation of implanted matrix to the host tissue. In addition, mitigating poststroke inflammation and subsequent scar formation is crucial in scaffold integration, since astrocytic scar and associated chondroitin sulfate proteoglycans (CSPGs) may seal off the foreign object (in this instance the implant) from normal brain tissue and limit any interaction such as cell migration and axon extension. This fact highlights the importance of hydrogel biocompatibility to avoid a sustained inflammatory response and ensuing glial scar formation [123]. Angiogenesis is another landmark of integration that interconnects the host tissue to implanted matrix, supports growth of implant by providing oxygen and nutrients, and establishes trails for cell migration. In the following sections, we elaborate on biomaterial-based strategies to address different components of integration that improve cell therapies for stroke.
Inflammation, Glial Scar, and Foreign Body Reaction
Any nondegradable substrate in the brain leads to a constant activation of an inflammatory response that isolates the “foreign object” by forming an astrocytic scar [124]. This scar is a counter-integration phenomenon since it serves as a barrier for cell migration and axon growth through the scaffold-tissue interface [125]. Biopolymer hydrogels have several characteristics that improve their biocompatibility and integration in the brain.
Mechanical trauma during scaffold implantation causes an immediate inflammatory response that ultimately leads to the foreign body response against implants [126, 127]. This could be avoided in hydrogels by employing minimally invasive methods of gel injection. In addition, the high water content of hydrogels further improves their biocompatibility [61]. The size and shape of hydrogels are additional biophysical parameters that determine surface area available to inflammatory cells and therefore affects biocompatibility [127, 128]. The stiffness of the implanted matrix is another physical factor contributing to prolonged inflammation and implant isolation. Astrocytes and microglia, the main cells involved in inflammation in the brain, are primed by stiff substrates to produce proinflammatory molecules such as IL-1β and toll-like receptor (TLR)-4, respectively [123]. Implantation of hydrogels with elasticities higher than the brain’s values incites inflammation in the early phase following implantation that leads to astrocytic scar in chronic phase [123]. This indicates scaffolds similar in stiffness to the brain will optimize differentiation in encapsulated stem/progenitor cells [110] as well as promoting integration to the surrounding brain tissue. Another solution to render hydrogels biocompatible is to make them degradable in the brain. Hydrogels provide support for encapsulated cells early after implantation and following growth, differentiation, and deposition of their own matrix, hydrogel is degraded by encapsulated or host tissue cells. Examples are HA [88] and collagen [66] gels that are degraded by cellular hyaluronidase and collagenase, respectively. It is important, however, to determine that hydrogel degradation products are nontoxic and cleared away.
In the pursuit of more biological solutions to improve biocompatibility, hydrogels have been loaded with a variety of anti-inflammatory factors (for details see “Trophic Support and Protection from Inflammation”). Chondroitinase ABC, a digestive enzyme for CSPG molecules, has also been used in implantation of neural stem cell (NSC)-loaded poly-ε-caprolactone scaffold to promote migration of NSCs into the injured CNS tissue [129]. In addition, high molecular weight HA gels through their anti-inflammatory effect can attenuate scar formation and improve integration of encapsulated cells [88]. This indicates biopolymer scaffolds, through their physical, chemical, and biological properties, attenuate inflammation and scar formation, and therefore promote integration of transplanted cells to the host brain tissue.
Angiogenesis
Oxygen and nutrients diffuse up to 150–250 µm from capillaries [130]. Therefore, any poststroke tissue reconstruction beyond these dimensions necessitates establishment of an environment with accessibility to blood perfusion. This requires formation of blood vessels reaching into the transplanted matrix. In fact, there is a correlation between transplanted cell survival and extent of new vessel formation [131]. Angiogenesis involves activation of endothelial cells by proangiogenic factors, such as vascular endothelial growth factor (VEGF), PDGF, FGF, hepatic growth factor (HGF), angiopoietin-1, and transforming growth factor-β or TGF-β. Endothelial cells then branch off from existing capillaries, proliferate, and migrate into the transplanted matrix [132, 133]. For successful angiogenesis, the transplanted matrix should support survival and proliferation of endothelial cells. In addition, the matrix has to allow remodeling by endothelial cells, forming cord-like structures which will mature into capillaries, and subsequently veins and arteries.
Hydrogels as highly hydrated and porous substrates are supportive for endothelial cell migration. A network of interconnecting and communicating pores facilitate angiogenesis and obviates the need for matrix digestion by endothelial cells. Angiogenesis could be further promoted by enriching the hydrogel environment and introducing elements of ECM matrix to the hydrogels, such as fibronectin [134] or fibronectin-derived synthetic polypeptide motifs [135]. Incorporation of slow-releasing proangiogenic factors, such as VEGF or bFGF, is another means to potentiate formation of new vessels inside a hydrogel matrix [136]. Migration of endothelial cells and remodeling of matrix involves secretion of MMPs, such as MMP-2 and MMP-9. Therefore, hydrogels with motifs recognized and cleaved by MMPs will have an additional proangiogenic effect. This could be achieved by including MMP-sensitive cross-linkers. A study combined both strategies by including VEGF tethered through a MMP-sensitive linkage and showed improved angiogenesis [118]. Some biopolymer hydrogels particularly support formation and stability of new vessels. HA gels are an example: While high molecular weight HA leads to endothelial cell quiescence, oligomeric HA, produced from degradation of HA gels, promotes proliferation and migration of endothelial cells by interacting with HA cells receptors, such as CD44, hyaluronan-mediated motility receptor (RHAMM), and TLR-4 [137]. In fact, HA hydrogel has been shown to promote angiogenesis upon implantation to the brain [138].
Inclusion of NSPCs adds to a proangiogenic benefit of hydrogels. NSPCs perceive lack of oxygen and nutrients by expressing the transcription factor hypoxia-induced factor-1 that leads to increased secretion of VEGF [139]. Secretory support of angiogenesis by NSPCs could be enhanced when they are encapsulated within a hydrogel with 40 kPa (compressive modulus) [111]; this is another example of how mechanical properties contribute to regenerative potential of cell scaffolds. NSPC proangiogenic effects can be further enhanced by inclusion of endothelial cells in a co-transplantation approach. In fact, this combination of cells encapsulated in PLGA gels has improved angiogenesis at the center of traumatic CNS injury and promoted reestablishment of the blood–brain barrier [140]. The application of biopolymer matrices with optimized mechanical (i.e., porosity and elasticity), biochemical (i.e., degradability, inclusion of ECM elements and growth factors), and cellular composition will enhance formation of new vessels, which in turn contributes to survival and integration of transplanted cells.
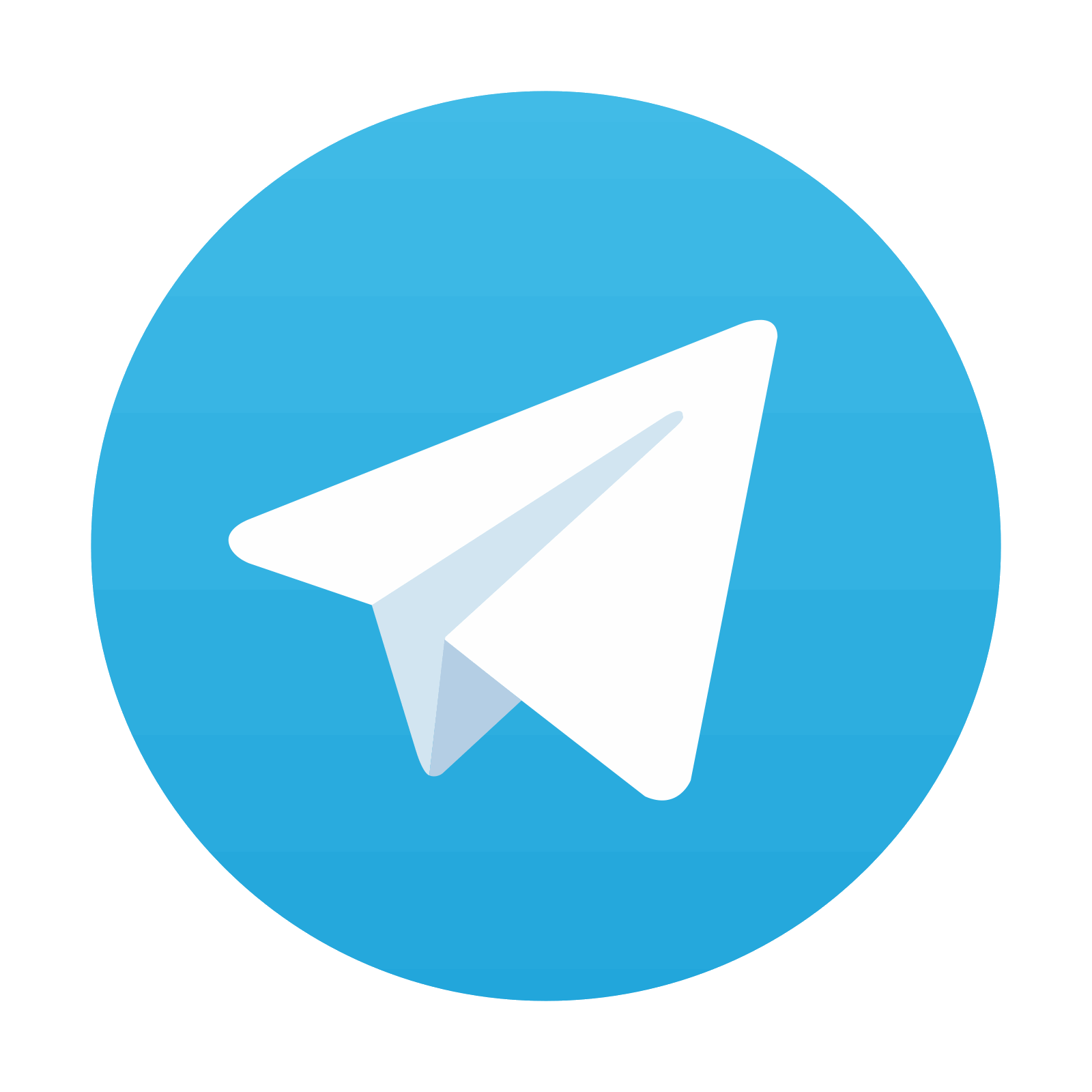
Stay updated, free articles. Join our Telegram channel
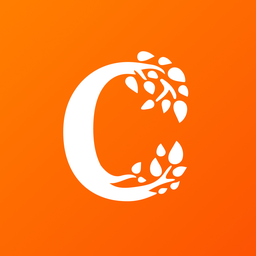
Full access? Get Clinical Tree
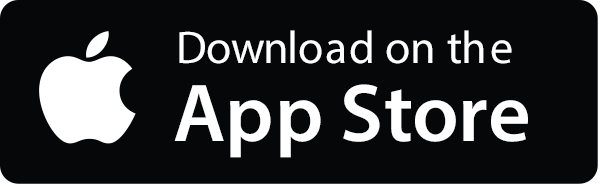
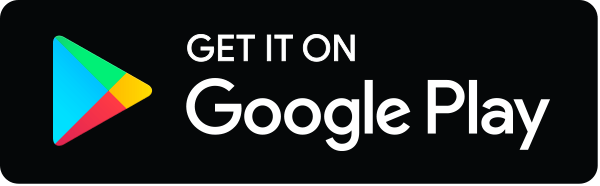