4 Biomechanics of the Lumbar Intervertebral Disc Michael N. Tzermiadianos and Avinash G. Patwardhan A disc comprises three distinct parts: the nucleus pulposus (NP), the anulus fibrosus (AF), and the cartilaginous end-plates. The nucleus is composed of a loose network of fibrous strands that lie in a translucent mucoprotein gel containing various mucopolysaccharides. In a healthy young disc, the water content of the nucleus ranges from 70 to 90%. The water gives the tissue very low rigidity so that it can deform easily in any direction and equalize the stress applied to it.1 The nucleus fills 30 to 50% of the total disc cross sectional area, and is located more posterior than central. The anulus gradually becomes differentiated from the periphery of the nucleus and forms the outer boundary of the disc. The anulus is made up of a series of 15 to 25 concentric lamellae.2 The fibers within each lamina are arranged in a helicoid manner angled at 30 degrees with respect to the endplate.2 The fibers of adjacent lamellae have similar arrangements, but run in opposite directions with the fibers of one layer angled to the right and the fibers of another layer angled to the left. The anulus is well suited to resisting torsion due to the characteristic orientation of the fibers in each layer. Because of this opposite orientation, torsional movements generate tension in half of the collagen fibers in the anulus, whereas the other fibers tend to become slack.3 Fiber strains rarely exceed 6% under physiologic flexion and extension moments and 8.5% under physiologic axial rotation. The intervertebral disc (IVD) alone provides most of the compressive stiffness of the motion segment, whereas ligaments and facets contribute significantly to resisting bending moments and axial torsion. The annular fibers are attached to the cartilaginous end-plate in the inner zone, whereas in the outer zone they are attached directly to the osseous endplates. This attachment to the bone through Sharpey’s fibers is significantly stronger than the other more central attachments to the cartilaginous endplates. The anulus contains the nucleus, surrounding it like a strong thick membrane. When the disc is compressed, the pressure inside the nucleus increases, generating a tensile hoop stress in the restraining anulus, thus maintaining the IVD height. Hoop stress decreases from the inner lamellae of the anulus to the outer lamellae. Cadaveric experiments have shown that a compressive force of 2000 N stretches the collagen fibers on the outer anulus by less than 2% and causes the anulus to bulge radially by 0.4 to 1.0 mm.4 As the preload increases from 250 N to 4500 N, the height of a motion segment is reduced by 0.9 mm. Approximately half of the height reduction can be attributed to the endplates bulging into the vertebral bodies.5,6 The anulus also resists compression directly; therefore, compressive stresses are distributed almost evenly throughout the entire disc area in a young, nondegenerated disc.7 The natural human disc has 6 degrees of freedom, allowing independent angular motion about, and independent translation along each of the three anatomic axes.8 Being a fibrocartilaginous joint, the disc offers resistance to both angular and translational motions. The kinematic properties of the disc, and those of the whole motion segment, can be assessed by measuring the range of motion (ROM) and the parameters that assess quality of motion. ROM refers to the total amount of motion between the motion endpoints. The average ROM in the flexion-extension in healthy young men, measured using stereo radiographs was ~14 degrees at most spinal levels.9,10 ROM was ~4 degrees in lateral bending, and 1.5 degrees in axial rotation. The L5-S1 segment showed significantly lower in vivo ROM compared with the other segments. The term quality of motion refers to the characteristic kinematic signature of the disc (or a spinal segment), and characterizes the pattern of motion as opposed to its magnitude. When a spinal segment is subjected to flexion and extension moments, the load-displacement curve has a characteristic sigmoidal shape, with concavity toward the load axis (Fig. 4.1). Such a curve implies that the disc and the surrounding ligamentous structures provide little resistance (i.e., low stiffness) at low loads, but at higher magnitudes of load the stiffness is increased. Thus, it provides flexibility at low loads and stability at higher loads. Two commonly used parameters to characterize this curve pattern are neutral zone and stiffness in the high flexibility zone. Neutral zone, expressed in degrees, is calculated as the difference in the segmental angle between the loading and unloading curves at 0 Nm bending moment.11 The high flexibility zone is the region around the neutral posture in which the osteoligamentous spine can move with negligible resistance. The stiffness in the high flexibility zone is calculated using slopes of the linear portion of the load-displacement curve around the neutral posture.12 These concepts of neutral zone and stiffness also apply to the discussion of quality of motion in lateral bending and axial rotation. The characteristic shape of the load-displacement curve, and therefore the values of neutral zone and stiffness are affected by compressive preload and disc degeneration. Fig. 4.1 Load versus displacement curve of a lumbar segment in flexion-extension. Another measure of the quality of motion of a spinal segment is the location and orientation of the helical axis of motion (HAM) as the segment undergoes motion in response to physiologic loads. The quality of segmental motion in the sagittal plane can be assessed by the location of the center of rotation (COR), which is the intersection of the HAM with the midsagittal plane.13–15 The COR location can be compared between healthy and degenerated segments to assess the difference in the quality of flexion-extension motion. In a recent in vitro study,16 the COR in flexion-extension for healthy L3-L4 and L4-L5 segments calculated using the flexion and extension endpoints was located close to or slightly posterior to the midpoint of the superior endplate of the inferior vertebra. In contrast, in the L5-S1 disc space, the COR was located ~4–5 mm posterior to the midpoint of the S1 endplate (Fig. 4.2). The instantaneous centers of rotation (ICR) calculated for incremental motions during flexion-extension in the relatively healthy segments tended to remain stationary as the segments moved through the ROM.16 These experimental results of COR in intact cadaveric specimens match closely with the in vivo results reported by Pearcy and Bogduk17 and Schneider, Pearcy, and Bogduk.13 In all three studies,13,16,17 the COR was located close to the superior endplate of the inferior vertebra of the L4-L5 and L5-S1 segments, unlike the in vitro results of Zhao et al,18 who reported the location of the COR in cadaveric specimens to be 30 mm caudal to the midpoint of the disc. Zhao et al’s measurement puts the COR near the inferior endplate of the inferior vertebra, a location too distal when compared with the results reported in vivo. It should be noted that the location of the COR depends on the loads used in creating the motion. A combination of flexion moment and anterior shear would result in a COR that is located more distal than that for motions created by pure moments. Fig. 4.2 Locations of flexion-extension centers of rotation for healthy lumbar segments. Loads on the human spine are shared by the osteoligamentous tissues and muscles of the spine. Tensile forces in the paraspinal muscles, which exert a compressive load on the spine, balance the moments created by gravitational and external forces. Because these muscles have a small moment arm from the spinal segment, they amplify the compressive load on the osteoligamentous spine. The preload, produced by muscles, can be considered an “external” compressive load that acts on the spinal segments in vivo during different activities of daily living. The mechanical response of healthy, degenerated, or injured spinal segments will be influenced by this preload. The internal compressive forces on the ligamentous spine have been estimated for different physical tasks using intradiscal pressure and electromyographic data in conjunction with three-dimensional biomechanical models. The compressive force on the human lumbar spine is estimated to range from 150–300 N during supine and recumbent postures to 1400 N during relaxed standing with the trunk flexed 30 degrees. The compressive force may be substantially larger when holding a weight in the hands in the static standing posture, and even more so during dynamic lifting. In healthy individuals, the spine sustains these loads without injury or instability.19–21 The IVD is the major load-bearing element in axial compression and flexion. In the young healthy spine, the disc transfers ~80% of the compressive load applied to the motion segment.22 As load is applied to the healthy disc, forces are distributed equally in all directions from within the nucleus, placing the anulus fibers in tension. The collagen fibers of the anulus are well suited to resisting tension. The pressure in the nucleus causes the lamellae of the anulus to bulge outward, stretching the fibers in the anulus. Resistance of the fibers to tensile loading then allows the anulus to contribute to compressive load sharing. Measurements in young, healthy discs using stress profilometry show that most of the disc is under uniform load and because the stress is equal in the vertical and horizontal directions (isotropic), the nucleus behaves as a fluid.23 Experimental and finite element studies have shown that a compressive load applied to a healthy disc is shared by both the NP and the AF.24 Adams et al25 showed that when discs are subjected to compressive loading in the neutral posture they generally exhibit a small peak of compressive stress in the posterior anulus and a fairly even compressive stress throughout the nucleus and anterior anulus. In extension, the size of the peak in the posterior anulus increases, whereas moderately flexed postures usually distribute stresses evenly across the disc. In full flexion, stress peaks appear in the anterior anulus but are rarely as high as those in the posterior anulus are in full extension.25 Posture also affects the hydrostatic pressure in the nucleus. Under compressive preload of 500 N, the nucleus pressure is 40% less in 4 degrees of extension than in the neutral posture, reflecting the increased load sharing by the facets in extension.25 Nucleus pressure rises by 100% in full flexion because flexion stretches the ligaments of the neural arch, creating tension that compresses the disc. If the neural arch is removed, the ligamentous tension decreases, and the nucleus pressure increases only by 38% in flexion and by 8% in extension.25 Loading effects on the lumbar spine not only depend upon load magnitude but also upon loading rate and duration.26,27 This dependency occurs because of the viscoelastic behavior of the IVD.22 Viscoelasticity is defined as the time-dependent response of a material to rate of loading or deformation and is linked to the absorption of energy by the disc. Viscoelastic materials exhibit hysteresis, which is a measure of the loss of energy when a structure is subjected to repetitive load and unload cycles. Hysteresis can be thought of as a protective mechanism as this mechanism is mainly responsible for the discs absorbing the shock energy from the sacrum to the skull when a person lands on his or her feet. Hysteresis may vary according to the load applied as well as the spinal level and degree of degeneration. Hysteresis is also decreased when the same disc is loaded a second time, reaching a steady state after a few cycles.28 Viscoelastic behavior of the disc comes from two sources: the inherent viscoelasticity of collagen fibers and their interaction with the proteoglycan matrix, and the fluid flow within the disc under load. The outflow of interstitial fluid under loading causes approximately a 20% reduction in height and the volume of the disc.29 Intradiscal pressure has been shown to decrease with creep loading,30 rendering the tissue less resistant to bending.26 When loading is removed, the disc reabsorbs water and recovers from the deformation. As compared with compression, the lumbar discs appear less well suited to resist prolonged loading and low-frequency cyclic loading in shear. Biomechanical studies have reported that load transfer in compression occurs via the production of high disc pressures; whereas in shear, the mechanism appears to be via the AF without the development of significant disc pressure.31,32, Thus, the stiffness in shear under static and dynamic loads is significantly smaller than the values in compression.33 The stress relaxation in shear is also significantly smaller than the values in compression.33 This implies that under prolonged shear loading, the disc will retain a higher proportion of the applied shear load as compared with the proportion of the compressive load retained by the disc when loaded in pure compression. Conversely, under a low-frequency cyclic loading environment, the ability of lumbar discs to dissipate the dynamic energy (hysteresis) is significantly smaller in shear than in compression.33 These findings suggest that the disc is susceptible to injury when loaded in shear. Disc degeneration is associated with biochemical, morphologic, and material changes. A loss of proteoglycans, and hence loss of hydration, particularly in the nucleus is considered the principal biochemical sign of degeneration.34 The loss of water content from the nucleus results in a loss of disc height. The nucleus becomes progressively more fibrous and opaque, with increased pigmentation. The demarcation between the anulus and the nucleus becomes less distinct, and delamination (separation of adjacent laminae) of the anulus occurs. Delamination leads to the development of concentric tears in the AF.35 These annular tears increase in size and coalesce to form radial fissures. The radial fissures then expand and extend into the NP, disrupting the disc structure. Radial fissures and cracks in the anulus can form cavities within the disc.36 In a cadaveric study, Krismer et al37 reported that reduced disc height was consistently associated with the presence of fissures in the anulus. With disc dehydration and narrowing of the disc space, the nucleus is no longer able to exert a hydrostatic pressure on the anulus, meaning the annular fibers of the disc are no longer subjected to the same tensile stresses, as they would be in a healthy disc with a hydrated nucleus. Instead, the anulus in a degenerated disc under compression is more likely to directly bear the axial load from the vertebra above, resulting in an inward bulging of the inner anulus and accelerated delamination.38 Mechanical, chemical, age-related, autoimmune, and genetic factors have been implicated in the pathomechanisms of disc degeneration.39 Considering the limited repair capacity of the IVD, accumulative structural damage is believed to contribute to disc degeneration. Experimental studies have shown that compressive load generally leads to failure of the endplate or the vertebral body but cannot produce disc herniation. The disc bulges circumferentially after compressive loading with no propensity for posterolateral protrusion.40 Axial rotation is likely a more important injury-causing load as proposed by Farfan et al.41 The IVD provides ~40 to 50% of the torsional strength, whereas the remaining strength is attributed to the posterior elements and the interspinous ligaments.41 Axial rotation, especially in combination with flexion when the facet joints open and offer less constraint to rotation,22 can lead to delamination and annular tears that can initiate the disc degeneration process. Depressurization of the nucleus reduces the intervertebral space height, subjects the anulus to more compressive load and allows more rotational flexibility because of the collapsed annular fibers and the capsular laxity of the facets that follows facet subluxation. Repeated loading in flexion has also been implicated in disc degeneration.42 Other mechanical encumbrances implicated in disc degeneration include acute hyperflexion injury,43 repetitive hyperextension injuries,44 and vibration.45 However, it must be noted that establishing a cause and effect relation in disc degeneration is difficult. Structural disruption is accompanied by cell-mediated changes, and progressive biochemical changes alter the structural integrity. Therefore, it is unclear whether mechanical disturbances or biochemical changes initiate the degenerative cascade. As narrowing of the disc space occurs, the zygapophyseal joints undergo subluxation until the tips of the inferior facets impinge on the inferior lamina. This causes an increase in the load transmission by the facets. Increased peak pressures caused by the increased loads within the facet joint may give rise to degeneration of the joint cartilage. Thinning of the cartilage may cause capsular ligament laxity and allow abnormal motion or hypermobility of the facet joints. Cartilage degeneration seems to further increase the segmental movements that already were increased with disc degeneration. As degeneration progresses, the abnormal pressure and focal degeneration of the facets give rise to bony hypertrophy and osteophytes with a subsequent decrease in segmental mobility. The effect of disc degeneration on segmental ROM depends on the degree of disc degeneration. According to the degenerative cascade proposed by Kirkaldy-Willis and Farfan,46 as degeneration increases the spinal segment progress from the dysfunction to the instability phase, and finally to the restabilization phase. Cadaveric studies have confirmed that segmental motion tends to increase with increasing severity of disc degeneration. However, a decrease in segmental motion has been found at the highest grade of degeneration.47,48 Flexion-extension radiographs have been traditionally used to diagnose spinal instability in vivo; however, the results have been inconsistent. Morgan and King49 observed the presence of incomplete radial posterior tears in the lower lumbar discs and of anterior concentric fissures in the upper lumbar discs, and drew attention to the association between annular tears, radiographic signs of instability, and low back pain. Other clinical studies showed reduction of disc height to be significantly associated with reduced flexion-extension ROM,50 a finding that has also been reported in cadaveric studies.37,47 Mimura et al47 reported that ROM in flexion-extension showed a tendency to decrease, whereas lateral bending showed a significant decrease with degeneration. On the contrary, Krismer et al37 did not find a significant decrease in the lateral bending ROM with increasing degeneration. The differences between reports might be due to different specimen selection and inclusion of specimens with lateral osteophytes in some of the studies. Increased axial rotation with higher degrees of disc degeneration has been more consistently reported among different investigators.37,47,48,51 Kirkaldy-Willis and Farfan46 focused on anteroposterior lateral bending radiographs from which rotational deformity and lateral translation were interpreted as indicators of instability. Cadaveric studies have confirmed the importance of torsional instability in disc degeneration. Krismer et al37 reported that the ROM in axial rotation is increased in cases of severe degeneration. Furthermore they reported that in disc degeneration axial rotation is coupled with same-side lateral translation, a combination that was negligible in healthy segments. The authors also noted that reduced disc height in radiographs was associated with fissure formation in the disc. Schmidt et al52 also reported that the presence of a high-intensity zone in the IVD, a radiologic sign of radial tears, is associated with reduced stiffness of motion segments, especially in axial rotation. Other studies have reported that the change in axial rotation motion caused by disc degeneration is greater than the change in flexion-extension and lateral bending.47,48 These findings are in accordance with studies showing that the IVD plays a significant role in restricting rotation,7,51 although there is some controversy about whether torsion of the lumbar spine is restricted primarily by the apophyseal joints.51 ROM is not an accurate measure of instability caused by degeneration. A patient with a painful degenerated spine may have decreased motion, whereas a ballet dancer with extensive motion may not have any clinical symptoms of instability. Furthermore, the ROM measurement is affected by voluntary effort and thus motion limitation because of pain. In a biomechanical sense, instability is quantified in terms of a loss of stiffness or an increase in flexibility of a functional spinal unit. Panjabi53 postulated that increased laxity around the neutral posture would put increased demand on the spinal musculature to provide the stability needed during activities of daily living. In turn, increased muscle forces would increase the stresses in the spinal components and may contribute to pain.53 Furthermore, increased joint laxity may be associated with insufficient tension in the spinal ligaments and anulus fibers, both of which contain nerve endings that allow them to act as proprioceptive transducers. Lack of sufficient tension in the anulus fibers and ligaments may delay detection of sensory information needed to regulate muscle tension, thus contributing to dysfunction of the active stabilization system of the spine.54 The neutral zone and stiffness around the neutral posture (Fig. 4.1) are important measures of the stability of the spine. In vitro studies have shown that neutral zone increases with disc degeneration, particularly in axial rotation and anteroposterior shear motions. Therefore, the neutral zone is considered to be a more sensitive parameter than ROM in relation to disc degeneration or spinal injury.55 The neutral zone ratio, a quotient of the neutral zone and the ROM, increases in value with greater joint laxity. Mimura et al47 reported that although disc degeneration decreased ROM in flexion-extension and lateral bending, degeneration increased the intervertebral joint laxity around the neutral position as demonstrated by an increase in the neutral zone ratio. The neutral zone and stiffness in the high flexibility zone offer excellent in vitro tools to assess the effects of disc degeneration or injury; however, their value in the in vivo assessment in patients is yet to be demonstrated. The behavior of COR has also been used to assess the effect of degeneration on the quality of motion of a spinal segment. Although the instantaneous COR of the intact segments with mild degeneration tends to remain relatively stationary as the segments move through the ROM, discs with moderate to severe degeneration show substantially larger anterior and superior migrations.13,18,56–58 This shift of the COR is a reflection of the mechanical instability of these segments. Disc degeneration can significantly alter the normal load sharing between the components of a functional spinal unit. In vitro studies have shown that in a degenerative disc the nucleus becomes depressurized as a result of the reduction of water content and increased fibrosis.59 This has been confirmed by in vivo studies that showed that the intradiscal pressure was significantly reduced in a degenerated disc,60 and the decrease was in accordance with the degree of disc degeneration as estimated by magnetic resonance imaging (MRI).19 Nucleus depressurization results in an increasingly larger load transmission through the anulus, especially in the posterior portion.59 Furthermore, the principal area of load transmission is highly dependent on posture, with a more prominent increase of stress concentrations in the posterior anulus when the segment is extended.60,61 With disc degeneration, the posterolateral anulus is no longer acting in its role of a nucleus-retaining membrane, but rather as a region transmitting compressive stress. This is associated with inward bulging of the inner lamellae.62 In more advanced stages of degeneration with disc space narrowing, it is possible for the neural arch to stress shield the posterior anulus in extension, so that much of the compressive load is transmitted through the neutral arch and the anterior anulus.61 The facet joints in a healthy spine normally bear ~20% of the load, but when there is a loss of disc height due to degenerative changes facet load bearing can be as high as 70%.22 In the degenerated disc, the structure of the nucleus changes to a nonhomogeneous mixture of fragmented and condensed collagen, areas of fluid, and on occasion, areas of gas. Isolated fragments of the anulus or the cartilaginous endplate may add to the loose fragments in the disc.63 Disc pressure profilometry studies in cadavers have demonstrated irregular areas of abnormal spot loading.59 Similarly, in vivo studies have shown occasional stress concentrations in the nucleus and multiple stress concentrations in the posterolateral anulus.60 The multiple stress concentrations in the posterolateral anulus probably correspond to annular fissures or radial tears. Extensive variability of stress distribution has been shown even in discs showing the same degree of radiographic degeneration.60 This variability demonstrates that the radiographic or MRI appearance of a disc is not a direct indicator of its mechanical competence. Abnormal pressure profiles measured by in vivo stress profilometry have been shown to correlate with abnormal discograms. Increase in pressure in the degenerated disc is related to pain provocation.60 However, McNally et al60 demonstrated that pain is not associated with simple overloading of the disc. Evidence from in vivo stress profilometry suggests that it is the pattern of loading rather than its absolute magnitude that causes pain. Abnormal loading of the posterolateral anulus was a predicting factor for pain on provocative discography. Shear stress generated between areas of low load and areas of high load may also cause acceleration of degeneration and painful loading of the endplate. Similarly, the focal loading of the nucleus observed in a smaller percentage of investigated levels may also lead to pain. In this manner, pain provoked by discography may result from overloading particular areas by the injection of the discographic dye.60 The pain may be generated by pain-sensitive nerve fibers in the peripheral anulus, or by the abnormal loading of the vertebral endplate, which is highly innervated.64 Disc degeneration has also been associated with abnormal viscoelastic response.30 Water loss from the nucleus after creep loading reduces the hydrostatic pressure in the nucleus, resulting in a transfer of load from nucleus to anulus.30 Degenerated discs exhibit decreased compressive and shear stiffness under static and dynamic loads, greater initial deformation, and approach equilibrium at a more rapid rate compared with nondegenerated discs.33,65–68 The complex structure of the IVD allows mobility while offering resistance to angular and translational motions. The disc along with the surrounding ligamentous structures provides flexibility at low bending moments while offering stability at higher moments. In cadaveric studies, segmental ROM tends to increase with increasing severity of disc degeneration, with a decrease in segmental ROM at the highest grade of degeneration. On the contrary, in most in vivo studies disc degeneration is associated with a reduced flexion-extension ROM. Therefore, ROM may not be an accurate measure of instability caused by degeneration, which is better quantified in terms of an increase in the neutral zone or a decrease in the stiffness around the neutral posture as shown by in vitro studies. Increased laxity around the neutral posture theoretically puts increased demand on the spinal musculature to provide the stability needed during daily activities, thus increasing stresses in the spinal components. Increased joint laxity may also be associated with insufficient tension in the spinal ligaments and anulus fibers that may result in a delayed detection of sensory information from proprioceptive transducers, thus contributing to dysfunction of the active-neuromuscular stabilization system of the spine. Another reflection of the instability of degenerated lumbar segment is the substantially larger migration of the center of rotation as the segment moves through the ROM compared with nondegenerated or mildly degenerated segments where it tends to remain stationary. The biomechanical changes responsible for symptomatic disc degeneration are not clearly understood. In addition to the instability with the consequent abnormal muscle activation, altered load sharing between the components of a functional spinal unit, and abnormal spot loading and stress concentration in the nucleus and the posterolateral anulus have been hypothesized as factors contributing to pain. 4. Stokes IA. Surface strain on human intervertebral discs. J Orthop Res 1987;5:348–355 15. Panjabi MM, Krag MH, Dimnet JC, et al. Thoracic spine centers of rotation in the sagittal plane. J Orthop Res 1984;1:387–394 20. Nachemson AL. Disc pressure measurements. Spine 1981;6(1): 93–97 27. Hutton WC, Adams MA. Can the lumbar spine be crushed in heavy lifting? Spine 1982;7:586–590 42. Adams MA, Hutton WC. Gradual disc prolapse. Spine 1985;10(6): 524–531 58. Ogston NG, King GJ, Getzbein SD, et al. Centrode patterns in the lumbar spine. Baseline studies in normal subjects. Spine 1986; 11:591–595
Biomechanics of the Normal Lumbar Intervertebral Disc
Kinematic Properties
Load Transfer
Biomechanics of the Diseased Lumbar Intervertebral Disc
Disc Degeneration
Altered Kinematics
Abnormal Load Transmission
Conclusion
References
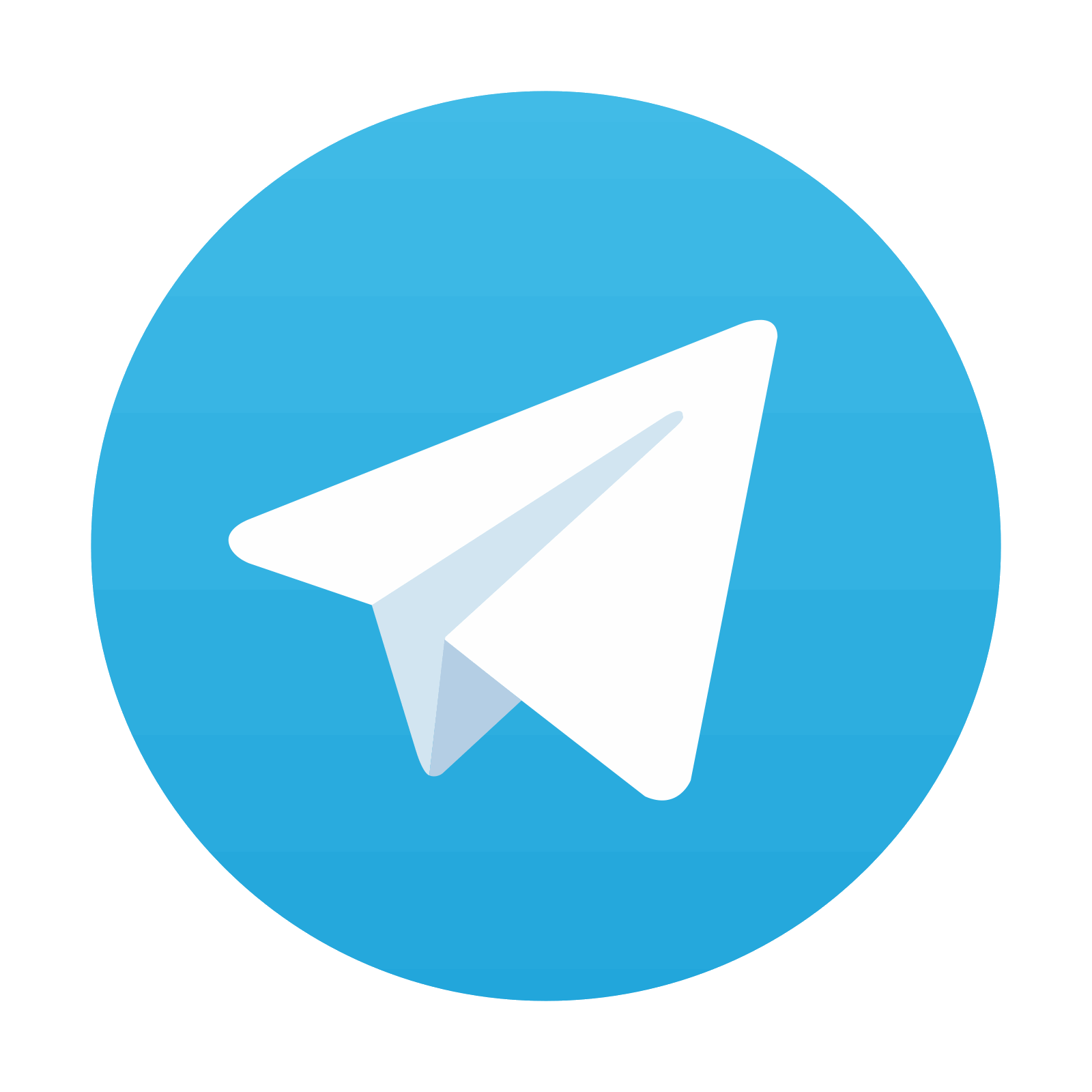
Stay updated, free articles. Join our Telegram channel
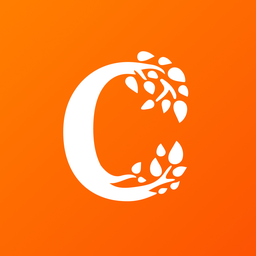
Full access? Get Clinical Tree
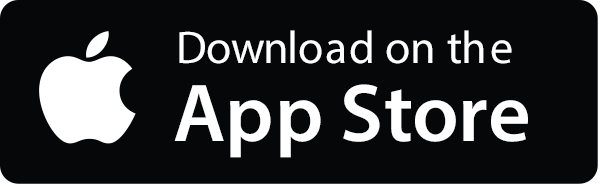
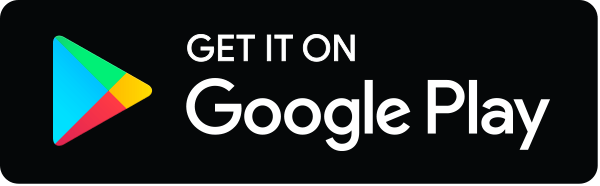