Blood–Brain Barrier Disruption
Abstract
The blood–brain barrier (BBB) is a dynamic system that separates the peripheral blood from the neural tissue. It is composed of endothelial cells connected through gap junction proteins and works together with various other cell types to create a unique microenvironment for proper neuronal function. BBB disruption, however, has been implicated in several neurological disorders including epilepsy. Various studies have demonstrated increased BBB permeability and albumin extravasation into the brain after prolonged seizures. Disruption of the BBB or direct application of serum albumin to the brain itself may also be sufficient to cause hyperexcitability. More recent research has focused on deciphering the mechanism of BBB dysfunction in the pathogenesis of epilepsy and several studies have suggested the involvement of the inflammatory transforming growth factor β (TGF-β) pathway. Early detection of BBB disruption may be clinically relevant and could be used to combat the development of epilepsy.
Keywords
Blood–brain barrier (BBB); tight junction; zonula occludens-1 (zo-1); biomarker; albumin; transforming growth factor β (TGF-β); extravasation
Overview
The blood–brain barrier (BBB) is a dynamic system that separates the peripheral blood from the neural tissue. It is composed of endothelial cells connected through gap junctional proteins and works together with various other cell types to create a unique microenvironment for proper neuronal function. BBB disruption, however, has been implicated in epilepsy. Various studies have demonstrated increased BBB permeability and albumin extravasation into the brain after prolonged seizures. Disruption of the BBB or direct application of serum albumin to the brain itself may also be sufficient to cause hyperexcitability. More recent research has focused on deciphering the mechanism of BBB dysfunction in the pathogenesis of epilepsy and several studies have suggested the involvement of the inflammatory transforming growth factor β (TGF-β) pathway. This chapter will review the current evidence for the involvement of BBB disruption in epileptogenesis and will also consider the clinical relevance of early detection of BBB damage.
History
The notion that the human body had an impermeable structure designed to protect the brain was first described by the London physician Humphrey Ridley (1653–1708) [1]. The first experiments to establish the existence of the BBB, however, were conducted by the German scientist and Nobel laureate, Paul Ehrlich (1854–1915) and his student Edwin Goldmann (1862–1913). In 1885, Ehrlich injected various dyes into the peritoneum of animals and noticed that almost all organs, except the brain and spinal cord, were stained [2,3]. He attributed the difference between the central nervous system (CNS) and other organs to different binding affinities to the vital dyes.
Goldmann furthered Ehrlich’s work by demonstrating that intravenous injections of trypan blue into dogs and rabbits turned the whole animal blue with the exception of the brain and CNS whereas injection of trypan blue into the brain ventricular system colored only the brain tissue and choroid plexuses [4,5]. These experiments confirmed that the lack of staining was not due to the lack of affinities for the dye and led to the hypothesis that the vehicle for transport was the cerebrospinal fluid (CSF) [2].
Two English scientists, Charles Roy (1854–97) and Charles Sherrington (1857–1952) [6] noted that the brain had an “intrinsic mechanism” to maintain the brain environment “without notable interference with the blood-supply.” They also determined that many lipid-soluble molecules could cross into the brain whereas many lipid-insoluble molecules could not [6]. The existence of a barrier between the blood and the brain, however, was first postulated a decade later by Max Lewandowsky (1876–1918). He discovered that intraventricular application of cholic acids or sodium ferrocyanide caused neurological symptoms whereas intravenous injections did not [7]. He thus concluded that the cerebral capillaries hindered certain compounds from entering the neuronal tissue and coined the term “blood–brain barrier” [1].
Until the 1950s, the hypothesis of a BBB was met with skepticism. It was suggested that the impermeability of the dyes were not due the presence of a brain barrier, but was instead due to their binding to albumin [1,2]. With the use of electron microscopy, several studies strengthened the hypothesis of the BBB and even described the structure and involvement of both endothelial cells and astrocytic endfeet in BBB formation [8–10]. It was not until 1967, however, that the site of the BBB was confirmed. Resse and Karnovsky [11] injected horseradish peroxidase (HRP) intravenously into mice and discovered that HRP did not pass the vascular endothelium in the cerebral cortex, thus determining the location of the barrier. Complimentary findings by Brightman demonstrated that intraventricular injections of tracer molecules are trapped between the glial and endothelial cells [12,13].
Structure and Function
The BBB is a highly regulated, multicellular interface between the peripheral circulatory system and the CNS [14,15]. The anatomical makeup of the BBB that surrounds the capillary lumen (Fig. 12.1) [16,17] is comprised of cerebral microvascular endothelial cells connected by tight junctions that prevent paracellular diffusion of water-soluble substances [17]. Astrocytic endfoot processes interact with the endothelial cells on the abluminal membrane (membrane of endothelial cells facing the brain) of the capillaries and play a role in maintaining BBB integrity. Pericytes are perivascular cells with elongated processes that intermittently surround the abluminal membrane of endothelial cells. More recently, pericytes have been shown to play a role in regulating cerebral blood flow [18]. Neurons may regulate local cerebral blood flow whereas microglia act as the immediate immune response within the brain. Pericytes, microglia, and neuronal projections closely associated with endothelial cells play a supportive role in the induction, maintenance, and function of the BBB [17,19,20]. The basement membrane, a thin layer of fibrous, extracellular matrix tissue, helps regulate cross talk between the various cellular components [15]. The basal lamina is a layer of extracellular matrix that, in part, makes up the basement membrane. Taken together, endothelial cells, astrocytes, pericytes, neurons, microglia, the basement membrane, and any other component of the brain parenchyma that communicates with these endothelial cells make up the neurovascular unit (NVU).

The cerebral endothelial cells form tight junctions at their margins which seal the aqueous paracellular diffusional pathway between the cells. Pericytes are distributed discontinuously along the length of the cerebral capillaries and partially surround the endothelium. Both the cerebral endothelial cells and the pericytes are enclosed by, and contribute to, the local basement membrane which forms a distinct perivascular extracellular matrix (basal lamina 1, BL1), different in composition from the extracellular matrix of the glial endfeet bounding the brain parenchyma (BL2). Foot processes from astrocytes form a complex network surrounding the capillaries and this close cell association is important in induction and maintenance of barrier properties. Axonal projections from neurons onto arteriolar smooth muscle contain vasoactive neurotransmitters and peptides and regulate local cerebral blood. BBB permeability may be regulated by release of vasoactive peptides and other agents from cells associated with the endothelium. Microglia are the resident immunocompetent cells of the brain. The movement of solutes across the BBB is either passive, driven by a concentration gradient from plasma to brain, with more lipid-soluble substances entering most easily, or may be facilitated by passive or active transporters in the endothelial cell membranes. Efflux transporters in the endothelium limit the CNS penetration of a wide variety of solutes (based on Abbott et al. [16]). Source: Reproduced with permission from Abbott NJ, Patabendige AA, Dolman DE, Yusof SR, Begley DJ. Structure and function of the blood-brain barrier. Neurobiol Dis 2010;37(1):13–25.
Early freeze-fracture electron microscopy studies were employed to gain insight into BBB structure [1,2,21,22]. Tight junctions, a key feature of the BBB, are arranged in a network of 6–8 anastomosing parallel strands, allowing them to be “very tight” and impermeable to most molecules [22]. In fact, the passage of molecules was restricted to a diameter of 10–15 Å [1,2]. The BBB exhibits a high transendothelial electrical resistance, which corroborated the extremely low ionic permeability of brain capillaries [23]. In addition to reducing paracellular diffusion of polar solutes, tight junctions contribute to the polarized (apical-basal) properties of endothelial cells [16]. Transmembrane proteins, including claudins and occludins, make up the junctional complexes. Claudins contribute to the high electrical resistance found throughout the endothelium whereas occludins regulate tight junction cytoskeletal activity [16,24]. Junctional adhesion molecules are involved in the formation and maintenance of tight junctions. Components of the BBB, including tight junctions, develop during embryonic stages and are well formed by birth [25–30].
In addition to the BBB, two other interfaces between the brain and epithelium exist. The blood–cerebrospinal fluid barrier is composed of epithelial cells of the choroid plexus facing the CSF. Here, epithelial cells may secrete CSF [31]. The arachnoid barrier completely seals the CNS from the rest of the body, although a relatively small amount of surface exchange between the blood and CNS occurs here. It is composed of the avascular arachnoid epithelium that underlies the dura. All three barriers play a role in modulating and regulating normal and pathological brain functions.
The BBB allows the brain to maintain a stable ionic and metabolic environment independent from the rest of the body. It has a variety of functions that help regulate and protect the brain, including responding to and passing signals between the blood and the CNS [15]. Specific ion channels and transporters found within the BBB help maintain ion balance that is idea for proper neuronal function. Because concentrations of ions such as sodium (Na+), potassium (K+), chloride (Cl−), and calcium (Ca2+) must be maintained within a very narrow range, this feature of the BBB is essential for neuronal health. In a similar fashion, the BBB prevents superfluous neurotransmitters, such as glutamate in the blood plasma, from entering the brain. The BBB also inhibits macromolecules and potential neurotoxins from entering the brain. For example, albumin can be damaging to nervous tissue and can cause glial scarring [17,32]. Finally, the BBB has specific transporters to ensure both the adequate supply of various essential nutrients and metabolites to the brain and the proper removal of waste products away from the brain [16,17].
BBB Disruption in Epilepsy
Disruption of the NVU is associated with a number of neurological disorders including stroke, Alzheimer’s disease, and epilepsy [33]. Prolonged seizures are associated with BBB dysfunction and a number of pathophysiological consequences including increased vascular permeability, reactive astrocytosis, leukocyte infiltration, inflammation, increased extracellular glutamate and K+ levels, and tissue hyperexcitability. While many studies have established a role for BBB damage in epilepsy, more recent research has been geared toward understanding whether BBB disruption is a cause or a consequence of the disease.
BBB Disruption as a Result of Epileptogenesis
Both human tissue data and animal models have been used to provide convincing evidence that BBB disruption may be a common pathological feature of epilepsy. BBB tracers such as Evans Blue, horseradish peroxidase (HRP), and fluorescein have been used to determine BBB permeability after seizure activity. Measurements of serum albumin or immunoglobulin (IgG) extravasation have also been used as indicators of a leak in the BBB. Dynamic changes in BBB permeability have been observed in patients with seizures and primarily during the epileptogenic period in animal models.
Human Tissue Studies
BBB disruption has been commonly found in tissue from patients with a number of neurological disorders including epilepsy, traumatic brain injury, and brain tumors. An early study (1984) found elevated serum albumin immunoreactivity around medium-sized blood vessels in the epileptic hippocampus compared to autopsy control tissue [34]. Van Vliet et al. [35] confirmed these results by demonstrating prominent albumin extravasation throughout resected hippocampi from patients with medically intractable epilepsy. Furthermore, albumin immunoreactivity was found in both neurons and astrocytes located around blood vessels and was particularly strong in patients who died during SE, suggesting a correlation between the time of seizure and magnitude of albumin extravasation [35]. Liu et al. [36] observed higher albumin and fibrinogen immunoreactivity in neurons, glial cells, and parenchyma in hippocampi from patients with either drug-resistant or drug-sensitive epilepsy compared to control tissue. Similar findings were seen in a variety of epilepsies.
Temporal lobe epilepsy (TLE) is the most common form of epilepsy in adults. In both the entorhinal cortex and hippocampus from patients with pharmacoresistant TLE, IgG leakage was observed in the parenchyma and in degenerating neurons [37]. Pyramidal neurons and interneurons were strongly labeled for IgG whereas dentate granule cells were faintly stained. A separate study confirmed IgG leakage and accumulation in neurons, possibly due to the loss of tight junctions [38]. In addition, vessel density was higher in tissue from patients with TLE than autopsy controls and this finding was positively correlated with seizure frequency [38].
Focal cortical dysplasia (FCD) is a cortical developmental malformation often associated with medically refractory epilepsy. In patients with FCD with balloon cells, hemosiderin deposits (an indicator of BBB impairment) were rare and no substantial albumin uptake into astrocytes was observed [39]. Although this study suggests that little to no impairment of the BBB occurred in patients with FCD, more studies need to be conducted to verify this finding.
An increasingly common way to measure BBB permeability is through the use of magnetic resonance imaging (MRI). Brain MRIs revealed increased BBB permeability in the majority of patients with posttraumatic epilepsy (PTE) compared to 25–33% of patients without seizures, an observation that could be found years following the trauma [40,41]. A separate study determined that a subset of patients with postconcussion syndrome have focal cortical dysfunction in conjunction with BBB disruption [42]. Since concussions are often related to cortical seizure activity, it is possible that BBB disruption played a role in the pathophysiology of seizure development and spread.
Gangliogliomas, characterized by dysplastic neurons and neoplastic astrocytes, are the most frequent tumor entity found in young patients with drug-resistant epilepsy. Schmitz et al. [39] found strong deposits of hemosiderin in ganglioglioma tissue. Albumin deposits in astrocytes were found in all gangliogliomas, but no correlation between albumin accumulation and duration of epilepsy was found [39]. In diffuse astrocytomas, on the other hand, neither hemosiderin nor astrocytic albumin deposits were seen in lesional or perilesional regions [39]. Although hemosiderin was present in tissue from patients with renal cell carcinoma brain metastases, almost no albumin uptake into astrocytes was observed [39]. WHO grade 1 dysembryoplastic neuroepithelial tumors exhibited both intralesional hemosiderin deposits and low levels of astrocytic albumin uptake [39].
Patients with vascular malformations, such as cavernous angiomas (CAs, intracerebral vascular malformation) or arteriovenous malformations (AVMs, abnormal blood vessels connecting arteries and veins), frequently have chronic focal epilepsy. Strikingly high levels of albumin were observed in surgically removed brain parenchyma surrounding CAs and AVMs [43]. Albumin accumulation was found to occur primarily in astrocytes.
Human tissue experiments often give information about the endpoint of a disease since they are usually conducted in chronically epileptic or postmortem tissue. Bolwig et al. [44], however, conducted a unique experiment where BBB permeability was examined after inducing electroshock seizures in patients undergoing electroconvulsive therapy for depression. Radioactively labeled Na+, Cl−, urea, and thiourea were injected into the internal carotid artery while a continuous series of blood samples were collected from the venous catheter before, during, and after seizures. During seizures, a significant decrease in thiourea extraction and a significant increase in urea permeability-surface area products were detected; no other differences from resting measurements were observed [44]. In addition, cerebral blood flow and venous O2-tension increased during seizures. Postictal levels were similar to resting levels [44].
Although studying postmortem human tissue has commonly been employed, some caution should be exercised when interpreting the results. In some cases, patients may have been suffering from other illnesses. For example, in the 1984 study examining albumin immunoreactivity in epileptic brain tissue, one patient suffered from cardiac malformations and died from cardiac arrest, which is known to cause severe hypoxic nerve cell damage [34]. The compounding effect of this hypoxic episode must be considered when evaluating the findings. An additional drawback to human tissue data is the inability to study a time course of the disease. For this, we must turn to studies using animal models of epilepsy.
Animal Models
Some of the earliest studies on BBB disruption after seizures showed increased permeability of BBB after pentylenetetrazol (PTZ)-induced seizures in a variety of animal models [45–48]. PTZ-induced seizures in rabbits quickly led to bilateral Evans Blue leakage into the hypothalamus (except the mammillary bodies), the preoptic area, midbrain tegmentum, and in the cerebellum (Fig. 12.2) [49]. Within a half-hour of PTZ-injections in adult rats, BBB permeability (measured by Evans Blue) was increased in the left hemisphere, right hemisphere, brainstem, and cerebellum [50]. These rats also exhibited increased immunoreactivity of zonula occludens-1 (zo-1), the main tight junction protein, after PTZ-induced seizures [50].

(A) The brain exhibits intense leakage in hypothalamus, excluding the corpora mammillaria, in the inferior olives and in the cerebellar hemispheres. Tuberculum olfactorium and the cortex around the anterior sulcus rhinalis are also stained in this exceptionally heavily affected rabbit. (B) Dorsal view of the same brain as shown in (A). Extremely intense Evans Blue (EB) stain in the cerebellum. The fronto-dorsal cortex shows a faint blueish stain. (C) Coronal cut at the level of the chiasma opticum. Intense leakage in the preoptic area, which spreads into pallidum and claustrum. The caudate nucleus remains free of stain. (D) Coronal cut at the level of the tractus mammillothalamicus. The tracer is concentrated around the third ventricle and in the geniculate, medial and dorsal thalamic nuclei. No stain is present in the center median. (E) Distinct EB leakage in the inferior, but not superior, colliculus. Source: Reproduced with permission from Nitsch C, Klatzo I. Regional patterns of blood-brain barrier breakdown during epileptiform seizures induced by various convulsive agents. J Neurol Sci 1983;59(3):305–22.
Lee and Olszewski [51] demonstrated that electroshock increased BBB permeability in cats and rabbits, particularly in the thalamic and hypothalamic areas of the brain. Studies in the 1970s demonstrated that electroconvulsive shock enhanced BBB permeability [44,52–55]. Measurements of radiolabeled Na+ and Cl− as well as carbon labeled thiourea (14C-thiourea) and glucose (14C-glucose) demonstrated a decrease in glucose extraction during electroshock-induced seizures in rats; all other tracers remained unchanged [52]. Repeated electroshocks in rats resulted in Evans Blue staining or HRP extravasation throughout the brain [52,55]. This and similar electrical stimulation models of epilepsy are still employed today to study BBB permeability.
Six weeks after status epilepticus (SE) induced by electrical stimulation of the angular bundle, rats exhibited BBB leakage (measured by fluorescein) in limbic brain regions [56]. In a rat model involving tetanic stimulation of the hippocampus, albumin and Evans Blue extravasation were prominently observed in the parenchyma of the hippocampus, entorhinal cortex, piriform cortex, thalamus, and amygdala; staining was also seen in the septum and olfactory bulb, but not in the cerebellum or in the brains of control rats [35]. Extravasation was highest in the acute seizure phase (1 day after SE), was still observed in the latent phase (1 week after SE), and was nearly abolished in the chronic phase (4 months after SE). Evans Blue colocalized abundantly with a marker for neurons and, to a lesser extent, markers for astrocytes and microglia. Interestingly, a higher seizure frequency was related to a more permeable BBB [35].
A number of studies conducted in the 1980s demonstrated disrupted BBB after seizures induced by kainic acid, an ionotropic glutamate receptor agonist [57–60]. Interestingly, rats that had only mild seizures after treatment with kainic acid did not exhibit BBB disruption [60]. Rats with severe limbic seizures showed increased permeability to α-[14C]aminoisobutyric acid autoradiography throughout the brain from 2 to 24 hours after kainic acid injections; BBB appeared normal from 24 hours to 7 days after kainic acid treatment, except for a small residual amount found in limbic structures [60]. Within hours of either intraperitoneal (i.p.) or systemic administration of kainic acid, rats exhibited increased permeability in the thalamus and the forebrain [58,59]. Subcutaneous injections of kainic acid in rats, however, led to only a mild increase in permeability of cerebral vessels [57]. Only about one-third of rabbits treated through the ear vein with kainic acid exhibited BBB breakdown [49]. In those rabbits, Evans Blue was found throughout the frontoparietal cortex and occasionally in the hypothalamus and thalamus, the substantia nigra, periaqueductal gray, basal forebrain, and amygdala [49].
Intraamygdala kainic acid injections into mice led to increased albumin and IgG amounts in the ipsilateral hippocampus to injection 4–24 hours after SE [61]. Levels did not differ from controls at 7 or 21 days post-SE with the exception of elevated IgG extravasation in CA3 of epileptic mice at 21 days post-SE [61]. In contrast to the early PTZ-induced seizure studies, reduced immunoreactivity for zo-1 was found at 1 hour, 8 hours, and 21 days post-SE, although no differences in Western blot protein amounts were observed [61].
After a single i.p. injection of kainic acid, strong IgG immunoreactivity was observed in adult mice 12-hours posttreatment, but it was restricted to the hippocampus [62]. By 3 days posttreatment, BBB integrity was restored. Interestingly, aged mice exhibited a low level of basal IgG staining, potentially due to age-related attenuation of BBB integrity [62]. IgG immunoreactivity was found throughout the aged mouse brain 12 hours after kainic acid treatment, particularly in circumventricular regions, increased by 24 hours, and remained visible until 7 days after treatment [62]. Contrary to these findings, however, Han et al. [63] found that i.p. administration of kainic acid in mice exhibited a dose-dependent inhibition of Evans Blue dye extravasation.
Similar to the human PTE studies, MRI has been employed in animal models to measure BBB disruption. In the intrahippocampal kainic acid model of epilepsy in rats, both MRI involving T1 mapping and postmortem microscopic analysis of fluorescein leakage determined that BBB leakage was evident in both the acute (1 day post-SE) and the chronic (6 weeks post-SE) phase in the hippocampus, entorhinal cortex, amygdala, and piriform cortex [64,65]. BBB leakage was most evident during the acute phase in limbic brain regions. MRI performed with T2 and T1 weighted images at various time points before and after subcutaneous injections of pilocarpine (muscarinic receptor agonist) revealed BBB leakage in the thalamus 2 hours after pilocarpine treatment, which disappeared by 6 hours [66]. No leakage was observed in either the latent or chronic phase of epilepsy.
Several additional studies have shown BBB leakage in the pilocarpine model of epilepsy in rodents [37,39,66–71]. A spotty immunostaining pattern for serum proteins was observed within the first 2 hours after pilocarpine treatment in rats [72]. As early as 6 hours after pilocarpine treatment, patches of serum extravasation appeared in the substantia nigra pars reticulata, likely as a consequence of neuronal and glial cell damage; this effect persisted for several days [72]. A separate study showed an increase in serum S100β levels and Evans Blue signal when measured at the onset of SE [70]. Analogous to results seen in humans, IgG accumulation in pyramidal neurons was observed around dilated microvessels during the acute period (1–12 hours postinjection) in the rat pilocarpine model of TLE [37]. Leakage was very faint during the latent phase (2–14 days postinjection) and slightly increased during the chronic phase (21–60 days postinjection) of epilepsy.
The majority of the animal model studies of BBB dysfunction in epilepsy have been conducted in adult rodents. Young rats, however, have demonstrated increased BBB permeability in response to seizures. In both adult and p21 rats, BBB opening to [α-14C]-aminoisobutyric acid occurred 90 minutes after pilocarpine-induced SE in the globus pallidus, lateral septum, and in most thalamic nuclei [69]. Adult rats also exhibited significantly increased permeability in the medial amygdala, cingulate and piriform cortices, geniculate nuclei, and anterior and ventromedial hypothalamus. In p21 rats, permeability significantly increased in the caudate nucleus, medial septum, parietal and sensorimotor cortices, hippo- campal CA1, and posterior and anteromedian thalamus [69]. Although both p21 and adult rats exhibited increased BBB permeability, it was often higher in the adults.
Methylazoxymethanol acetate (MAM) is a DNA-alkylating agent that has commonly been used as a model of human developmental brain malformations. Rats treated with MAM exhibited serum albumin leakage in the malformed hippocampus. Interestingly, pilocarpine-induced seizures exacerbated BBB leakage [71], thus suggesting that seizures can lead to disruption of the BBB in the deformed brain.
Rabbits intravenously injected with the GABAA antagonist bicuculline exhibited generalized tonic-clonic convulsions which quickly resulted in Evans Blue extravasation in the pallidum and the midbrain (including substantia nigra, midbrain tegmentum, and periaqueductal gray) but not the preoptic area or the superficial layers of the superior colliculus [49]. Similar results were obtained in a separate study of bicuculline-injected rats; HRP was frequently found in the thalamus, pallidum, hippocampus, and medulla oblongata but was rare in the septum, periaqueductal gray, hypothalamus, and cerebellar cortex [73]. A more recent study has used a bicuculline arterial perfusion model in vitro with a preparation isolated from guinea pig brain that maintained physiological interactions between neuronal, glial, and vascular components [74]. Sixty minutes after pulse applications of bicuculline, cells demonstrated significant brain extravasation of FITC-albumin and the extent of BBB damage correlated to the time spent in seizure-like activity [74]. Decreased levels of zo-1 in endothelial cells were observed [74].
Methoxypyridoxine (MP) blocks GABA synthesis whereas methionine sulfoximine (MSO) inhibits the conversion of glutamate to glutamine. MP-induced seizures in rabbits resulted in Evans Blue extravasation into the temporal parts of the hippocampus, hypothalamus, and dorsal parts of thalamus [49]. MSO-induced seizures in rabbits resulted in selective Evans Blue extravasation in the mammillary bodies [49]. In both models, a seizure duration of 3–5 minutes was enough to cause BBB damage and longer seizures did not result in increased Evans Blue leakage over time.
Hyperthermia-induced seizures induced in animals share a common pathology to febrile seizures seen in humans. Rats with cortical dysplasia introduced to hyperthermia-induced seizures exhibited increased BBB permeability to Evans Blue and HRP within 30 minutes after seizure induction [75]. Pregnant rats exposed to γ radiation exhibited fluorescein extravasation in the brain after hyperthermia-induced seizures [76].
A variety of animal models have shown BBB leakage shortly after seizure induction, however, this damage may persist into the chronic phase of epilepsy. One major concern when interpreting epilepsy model data is that the chemoconvulsants themselves, rather that the seizures, may disrupt the BBB. Fortunately, the variety of epilepsy models available, including chemoconvulsants, electrical stimulation, and hyperthermia-induced seizures, may assuage this concern. Illustrating BBB disruption in a genetic epilepsy model may also be beneficial in addressing this concern.
Seizures as a Result of BBB Dysfunction
Strong evidence exists to suggest BBB dysfunction results after seizure activity. More recently, however, researchers have shifted their focus on the ability of BBB disruption itself to lead to epileptogenesis. To address this problem, a number of substances, including bile salts and mannitol, have been used to open the BBB. Direct application of albumin to the brain has also been employed to study the immediate effect of BBB leakage. Human data on BBB disruption leading to tissue excitability are limited, however, due to the rare need to damage an intact BBB in a patient. Animal models must be substituted to address this question.
Human Tissue Studies
Due to obvious ethical limitations, very few studies have been able to determine seizure susceptibility in humans after BBB disruption. A subset of patients (25%) with primary brain lymphomas who underwent a treatment involving osmotic disruption of BBB with mannitol followed by intraarterial chemotherapy immediately developed seizures [77]. When just intraarterial chemotherapy was administered in the absence of BBB disruption by mannitol, patients did not develop seizures.
Cavernous malformations, or dilated blood vessels characterized by multiple distended “caverns” of blood-vessel vasculature, have a propensity to lead to seizures in patients [78]. Morphological analysis of cavernous malformations in human patients revealed a lack of tight junctions at endothelial cells interfaces and the absence of astrocytic endfoot processes at the site of lesions [78]. Therefore, it is possible that the lack of a functional BBB may lead to red blood cells leaking into lesions and the surrounding brain, thus accounting for the tendency of patients with cavernous malformations to have seizures.
Animal Models
Early studies of BBB disruption established that bile salts could open the BBB in a concentration-dependent manner [79–81]. Seiffert et al. [80] established a model for focal disruption of the BBB in the rat by directly applying bile salts to the cortex. Exposure to bile salts resulted in activation of astrocytes and extravasation of serum albumin in the extracellular space that lasted for several days [80]. A more recent study verified that exposure to bile salts led to the formation of an Evans Blue–albumin complex as early as 1 hour after treatment [82]. MRI in vivo revealed early BBB disruption with delayed reduction in cortical volume (associated with a reduced number of neurons and increased number of astrocytes) in response to both cortical treatment with bile salts and treatment with serum albumin [83]. In vitro experiments demonstrated that epileptiform activity could be recorded in the majority of slices from animals up to several weeks after treatment with bile salts [80,81,83]. Direct perfusion of the rat cortex with either serum, denatured serum, or albumin produced similar results, therefore eliminating the possibility of a bile salt-specific effect [80,81]. Treatment with a solution containing serum levels of electrolytes, on the other hand, did not induce abnormal activity in slices [80].
Frigerio et al. [68] quantified the amount of serum albumin extravasated in the rat brain parenchyma 24 hours after pilocarpine-induced SE and injected those levels of albumin intracerebroventricularly (i.c.v.) into naïve rats. EEG analysis revealed that rats injected i.c.v. with albumin developed high-amplitude, high-frequency spiking activity within 15 minutes after injection whereas dextran-treated control rats showed EEG activity similar to preinjection baseline [68]. Three months after albumin injections, rats exhibited a significant decrease in afterdischarge threshold, suggesting long-term effects on hippocampal excitability [68]. More recent studies revealed that intraventricular administration of serum albumin in mice did not immediately result in seizures, but did induce spontaneous recurrent seizures after a latent period of 3–5 days in the majority of mice [84,85]. These studies provided direct evidence for the role of albumin extravasation in seizure susceptibility in rats.
Hyperosmotic mannitol has commonly been employed to reduce BBB integrity. Successful mannitol-induced opening of the BBB in pigs resulted in seizures [77]. EEG activity recorded from rats after mannitol infusion demonstrated paroxysmal electrical activity and, in a few instances, tonic-clonic seizures [86]. Interestingly, this activity was only observed in rats with successful opening of the BBB. In contrast, a more recent study found that mannitol infusion did not induce seizures in healthy rats [35]. Chronically epileptic rats that underwent tetanic stimulation of the hippocampus, however, had increased seizure frequency during and after 3 days of mannitol treatment [35].
When considering these data, it is important to take into account the compound used to open the BBB, the concentration, route of administration, and the species examined. In addition, assumptions on what may leak out of a damaged BBB should be limited. Kang et al. [87] demonstrated that the BBB opening to large molecules does not necessarily mean that the BBB will open to smaller ions. Future studies should focus on the role of BBB disruption in epileptogenesis and ascertain the long-term consequences relating to neuronal hyperexcitability.
Mechanisms for BBB Disruption
Theories on the mechanism of BBB disruption in epilepsy models began in the late 1970s when it was first observed that increased BBB permeability after seizures was associated with an increase in systemic blood pressure followed by vasodilation [55,88–90]. Regional differences in BBB barrier breakdown, however, did not always correlate with increased regional blood flow, suggesting other mechanisms may be at play [49]. Bolwig et al. [55] reported increased vesicular transport (pinocytosis) across the endothelial cells during seizures, noting that the vascular structure remained intact. Several studies supported this hypothesis [54,90–93] and expanded it to include regulation by neurotransmitters, such as serotonin, binding to receptors on endothelial cells of brain vessels during seizure activity [49,73,93]. A competing hypothesis was the temporary opening of endothelial tight junctions during seizure activity [73,94]. More recent studies have focused on angiogenesis, mechanistic pathways, or activation of the immune response after BBB disruption.
Rigau et al. [38] discovered BBB impairment and increased expression of vascular endothelial growth factor (VEGF) in both tissue from patients with TLE and tissue from rats shortly after pilocarpine-induced SE. A progressive increase in vascularization occurred during the latent and chronic phases in the rat pilocarpine model of TLE [38]. Although seizures are associated with robust vasodilation, spreading depolarization may be associated with vasoconstriction, increased metabolic demand, and reduced energy supplies to neurons [95,96]. This may lead to the failure of Na+, K+, and Ca2+ pumps and consequent spreading and maintenance of neuronal depolarization.
Many studies have implicated the mammalian target of rapamycin (mTOR) pathway in epileptogenesis [97–101], but only one has examined the role of rapamycin in seizure-induced BBB permeability. SE was induced in rats by electrical stimulations of the angular bundle. Rodents were then treated with either rapamycin or vehicle control once daily for 7 days, then once every other day until 6 weeks post-SE. Vehicle-treated animals displayed BBB leakage in hippocampal regions whereas rapamycin-treated rats had significantly reduced BBB permeability [99]. Rapamycin treatment also decreased the number of epileptic rats and the number of seizures per day, but did not alter seizure duration [99]. Thus, the mTOR pathway may participate in seizure-induced BBB disruption.
Strong evidence suggests that inflammation is involved in the mechanism for BBB disruption after seizures. Brain inflammation can alter synaptic signaling, plasticity, inhibitory transmission, BBB permeability, and consequently hyperexcitability [65,102]. In the i.p. pilocarpine model of epilepsy, seizures induced BBB leakage, elevated levels of vascular cell adhesion molecules, and enhanced leukocyte rolling and arrest in brain vessels in mice [67]. Damage to the BBB, however, was prevented by blocking the leukocyte-endothelial interaction. Interestingly, human data showed that leukocytes were more abundant in epileptic tissue than in controls [67], further suggesting a potential role for leukocytes in the development of epilepsy. The involvement of leukocytes in BBB damage and its contribution to seizure generation, however, is a matter of debate [103].
Several antiinflammatory treatments have been shown to reduce or prevent the breakdown of the BBB and the occurrence of seizures. Intravenous administration of interleukin-1 receptor antagonist (IL-1RA) diminished the onset of SE and BBB damage in the rat pilocarpine model [104]. Pretreatment with the antiinflammatory drug dexamethasone reduced the number of rats that developed SE, increased the latency to onset, abolished mortality, and protected against BBB damage [70]. In addition, glucocorticosteroids (dexamethasone or adrenocorticotropic hormone) reduced the number of seizures in pediatric epilepsy patients [70]. Importantly, patients with syndromes known to respond to glucocorticosteroids, including Landau-Kleffner, Lennox-Gastaut, Rasmussen encephalitis, and West, were excluded from this study. Bicuculline-induced seizure activity was associated with over-expression of interleukin-1β (IL-1β) in isolated guinea pig brains [74]. Anakinra, a selective IL-1 receptor type 1 (IL-1R1) antagonist, reduced the duration of the first seizure, prevented seizure recurrence, and resolved seizure-associated BBB breakdown [74]. Taken together, these studies imply that inflammatory mechanisms may contribute to both BBB breakdown and seizure generation.
Elevated expression of IL-1β, IL-1R1, tumor necrosis factor-α (TNF-α), and transforming growth factor β1 (TGF-β1) mRNA were observed in the amygdala, hippocampus, parietal, prefrontal, and piriform cortices 2 hours after amygdala kindling in rats [105]. TGF-β was also found to be upregulated for the first 3 weeks after electrical angular bundle stimulation-induced SE [106]. TGF-β is a cytokine that plays a role in intercellular communication and in several cell functions including the immune response, cell growth, and apoptosis. In the canonical pathway, TGF-β binds to serine-threonine kinase TGF-β receptors (TGF-βRs) which results in the phosphorylation of Smad, an intracellular mediator, by activin-like kinase (ALK). Subsequently, phosphorylated Smad (p-Smad) translocates into the nucleus and promotes transcriptome activity. Several animal studies have implicated the activation of the TGF-β pathway as a potential mechanism linking BBB disruption to the development of epilepsy [65,102,107,108].
The application of serum albumin is associated with astrocytic activation [85], uptake into astrocytes in vivo [81,82], and hypersynchronous epileptiform activity in vitro [80–83,109]. Other studies have demonstrated that in addition to astrocytes, albumin is also taken up by NG2 cells [110] and neurons [84,110], with the most prominent quantity of uptake found in NG2 cells. The use of selective inhibitors in vitro demonstrated that albumin endocytosis into astrocytes involved TGF-βR activation, caveolae-mediated endocytosis, and an additional unidentified pathway [82].
Albumin uptake may upregulate cytokines, inducing brain inflammation, and activate the TGF-βR pathway [65]. Perfusion of serum albumin into the somatosensory motor cortex of rats led to spontaneous seizures, increased levels of phosphorylated Smad2/3 2 days after treatment, and astrogliosis 7 days after treatment [82]. Losartan, an angiotensin II type 1 receptor antagonist that has been shown to inhibit TGF-β signaling, reduced the number of rats that developed epilepsy, decreased seizure duration, and prevented the increase in reactive astrocytes and p-Smad2/3 levels without affecting regional blood flow [82].
Activation of the TGF-β pathway in rat neocortical slices resulted in epileptiform activity, similar to that seen after application of albumin [109]. Co-immunoprecipitation revealed a direct interaction between albumin and TGF-βRII [109]. Assessment of Smad2/3 phosphorylation levels revealed that albumin activated this pathway in epileptogenic rats [109]. Studies on cultured cells in vitro confirmed the occurrence of Smad2/3 phosphorylation and determined that albumin activates ALK5 [82]. Taken together, these data suggest that albumin entering the brain after BBB disruption may trigger the TGF-β pathway and lead to neuronal hyperexcitability (Fig. 12.3) [82].

With a dysfunctional BBB, the extravasation of Alb into the brain’s extracellular space leads to its interaction with astrocytic TGF-β receptors, activation of TGF-β signaling, and secretion of TGF-β1, followed by neuronal hyperexcitability and unprovoked epileptic seizures. Alb, albumin; ALK, activin-like kinase; p-Smad, phosphorylated Smad; TGF, transforming growth factor. Source: Reproduced with permission from Bar-Klein G, Cacheaux LP, Kamintsky L, Prager O, Weissberg I, Schoknecht K, et al. Losartan prevents acquired epilepsy via TGF-β signaling suppression. Ann Neurol 2014;75(6):864–75.
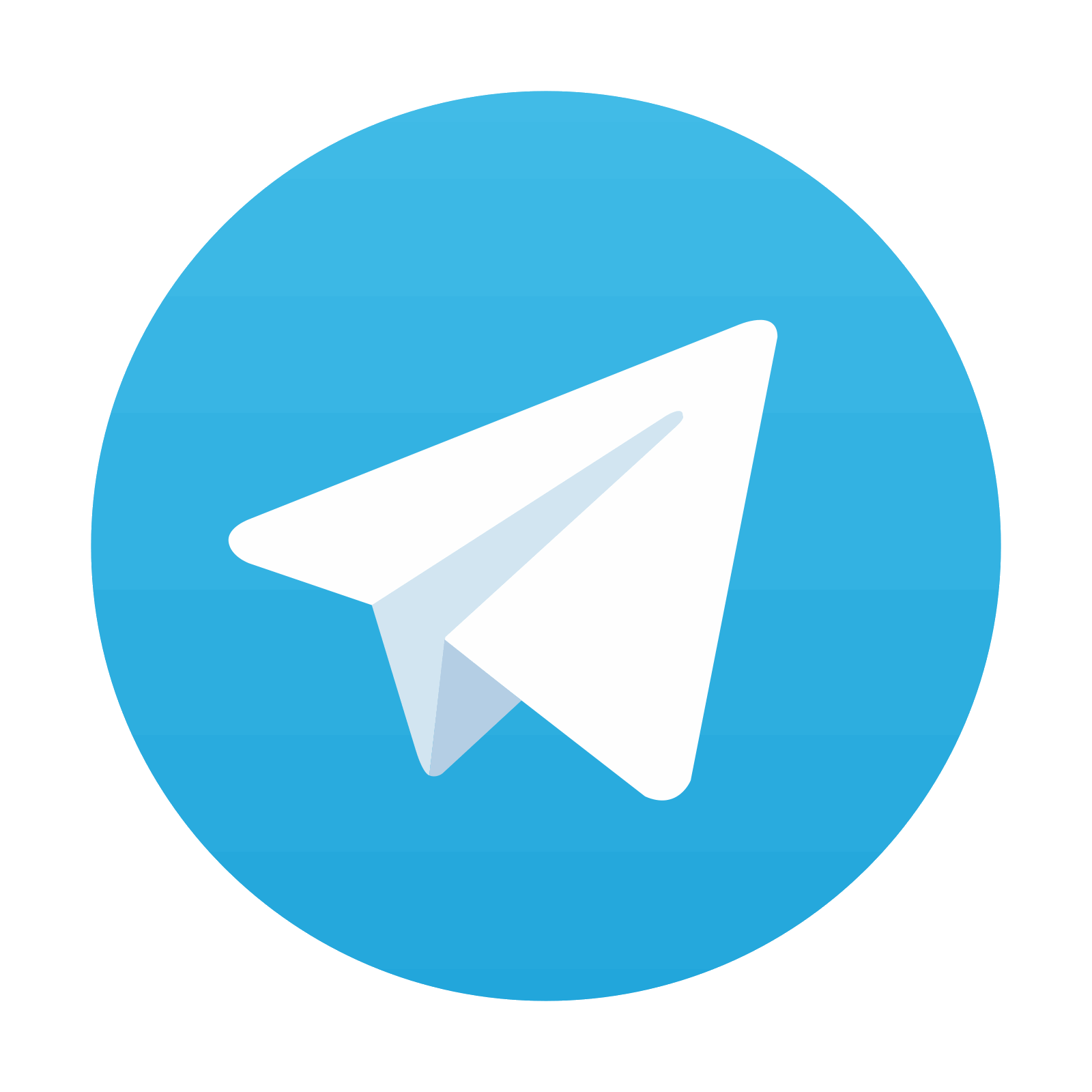
Stay updated, free articles. Join our Telegram channel
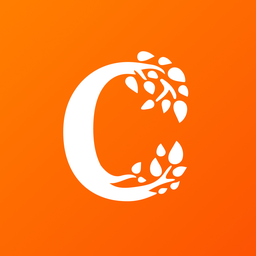
Full access? Get Clinical Tree
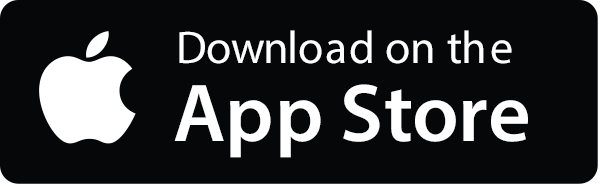
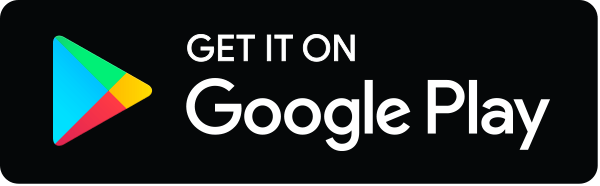
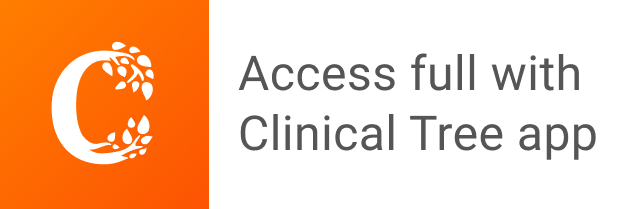