Gliotransmitters
Abstract
Glial cells were classically thought of passive “glue” that held neurons together. This view was challenged, however, with the use of new techniques, most notably patch-clamp electrophysiology and the use of fluorescent dyes, which has led to the discovery that astrocytes can participate in bidirectional communication with neurons and modulate synaptic transmission. Chemical signaling underlies this communication and strong evidence has demonstrated that neurotransmitters can be released from astrocytes in a process known as gliotransmission. We will focus on glutamate release, as it is the most studied gliotransmitter. At least six mechanisms of glutamate release from astrocytes have been identified: (1) calcium-dependent exocytosis; (2) reversal of membrane transporters; (3) glutamate exchange through the cystine/glutamate antiporter; (4) release through ionotropic purinergic receptors; (5) cell swelling-induced opening of anion channels; and (6) release through hemichannels. This chapter will discuss the evidence, controversy, and drawbacks for each mechanism of gliotransmission.
Keywords
Calcium; transporter reversal; cystine/glutamate antiporter; purinergic receptors; VRAC; hemichannels; glutamate; ATP
Overview
Historically, neurons were considered the primary cells responsible for brain function. This view has been challenged, however, by recent research proving the existence of astrocyte–neuron communication resulting in both the modulation of neurotransmission and the maintenance of brain homeostasis. Chemical signaling underlies this bidirectional communication and strong evidence has demonstrated that neurotransmitters can be released from astrocytes in a process known as gliotransmission. We will focus on glutamate release, as it is the most well-studied gliotransmitter. At least six mechanisms of glutamate release from astrocytes have been identified: (1) calcium-dependent exocytosis; (2) reversal of membrane transporters; (3) glutamate exchange through the cystine/glutamate antiporter; (4) release through ionotropic purinergic receptors; (5) cell swelling-induced opening of anion channels; and (6) release through hemichannels. This chapter will discuss the evidence, controversy, and drawbacks for each mechanism of gliotransmission.
A Neurocentric View
The classical view of glial cells was that they were primarily passive (serving as the “glue” to hold neurons together) whereas neurons were thought to be solely responsible for brain information processing [1]. Glia were largely ignored in terms of brain function and synaptic transmission. This was likely due to the fundamental difference between neurons and astrocytes: neurons are electrically excitable (can produce “all-or-none” action potentials that propagate through neuronal networks) whereas astrocytes are not. Because of this, astrocytes were often thought of as “silent” partners in the brain. This view has been challenged, however, with the use of new techniques, most notably patch-clamp electrophysiology and the use of fluorescent dyes, which has led to the discovery that astrocytes can modulate synaptic transmission.
A number of astrocytic properties have been discovered that led to the dismissal of the “passive” astrocyte theory and to our current understanding of the “active” astrocyte that plays a role in neurotransmission and neuronal physiology. Astrocytes in culture were found to exhibit depolarization in response to certain neurotransmitters, suggesting they possessed ionotropic receptors [2,3]. These cells respond to sensory input [4–8] and exhibit glutamate-induced calcium oscillations [9]. In addition, astrocytes play a role in metabolic support, uptake of potassium (K+), and clearance of neurotransmitters [10]. Most importantly, astrocytes engage in bidirectional communication with neurons [11,12], suggesting they are intimately involved in synaptic transmission.
The chemical synapse was traditionally thought to be composed of a presynaptic neuron and a postsynaptic neuron. The concept that a third component (the astrocyte) was involved gained popularity in the 1990s, although evidence for a communication system between neurons and astrocytes involving chemical transmitters was hypothesized well before then [13]. This led to the term “tripartite synapse” to highlight the direct role of astrocytes in synaptic function (Fig. 3.1) [14] (also see Chapter 2: Astrocytes in the Mammalian Brain).

(A) Electron micrograph showing a presynaptic (Pre) and postsynaptic (Post) terminal enwrapped by the astrocytic process (green) forming the tripartite synapse. (B) The close association of the astrocytic process with the presynaptic and postsynaptic terminals exerts crucial roles in clearing K+ ions that accumulate following neuronal activity, and in the uptake of the synaptic transmitter glutamate by the activity of plasma membrane glutamate transporters. Additionally, neurotransmitter release from presynaptic terminals can activate astrocytic metabotropic receptors, which induce the inositol (1,4,5)-trisphosphate (IP3)-dependent release of Ca2+ from internal stores, which in turn triggers the release of several neuroactive compounds (gliotransmitters) from these cells. Locations of astrocytic transporters and receptors in this figure do not necessarily represent their exact spatial distribution. Source: Reproduced with permission from Halassa MM, Fellin T, Haydon PG. The tripartite synapse: roles for gliotransmission in health and disease. Trends Mol Med 2007;13(2):54–63.
Astrocytes at the Synapse
Astrocytes are essential for proper synaptic development. Without them, few synapses would form and the ones that do would be functionally immature [15]. Imaging studies confirmed that astrocytes are organized into exclusive territories or “microdomains” with fine structures of appendages that are found either near or contacting synaptic clefts [16–19]. Through the use of serial electron microscopy and 3D analysis, it was estimated that about 57% of synapses in hippocampal area CA1 had astrocytic processes juxtaposed to them [20]. Overall, there was a large variation in processes surrounding the axon–spine interface, suggesting that astrocytes nonuniformly regulate synapses.
Astrocytes are crucial in maintaining the homeostasis of surrounding synapses. They supply energy metabolites, including citric acid cycle constituents [21–23], participate in the clearance and spatial buffering of extracellular K+ [24–27], remove glutamate from the extracellular space [28–31], and control extracellular levels and diffusion of neurotransmitters through astrocyte protrusion into the synaptic cleft [32–34]. Through these functions, astrocytes can modulate neuronal output. In fact, computational modeling has predicted that due to the dynamic relationship between neurons and astrocytes that ensheathe synapses, astrocytes may modulate the probability of vesicle release [35], optimize neural network performance [35,36], control the threshold value of transition from synchronous to asynchronous behavior among neurons [37], and lead to spike-timing-dependent plasticity at remote synaptic sites [38].
Bidirectional Communication Between Neurons and Astrocytes
The morphology and location of astrocytes allows them to both listen (through neurotransmitter receptors) and respond (G-protein-coupled receptors (GPCRs) and other intracellular pathways) to neuronal activity. Indisputable evidence has already demonstrated that astrocytes can respond to neuronal input, often with an increase in intracellular calcium. Emerging evidence, however, now suggests that astrocytes can discriminate between different inputs and that astrocytes can reciprocally respond to neurons with feedback signals.
Astrocytes Discriminate Between Different Inputs
Several studies have demonstrated that astrocytes have the ability to discriminate between different neurotransmitters and synaptic inputs. For example, astrocytes in hippocampal slices responded with calcium elevations to acetylcholine (ACh), but not to alveus stimulation-induced glutamate release, despite the presence of both cholinergic and glutamatergic axons [39,40]. Interestingly, astrocytes are able to integrate these inputs and can react to synaptic activity with a nonlinear input–output response [1]. Evidence for this has come from experiments involving visual, olfactory, and somatosensory stimulation.
Astrocytes in the primary visual cortex have various receptive-field characteristics similar to those seen in neurons, including spatially restrictive receptive fields, orientation tuning, and spatial-frequency tuning [41]. Astrocytes, however, had sharper orientation and spatial-frequency tuning than neurons and responded to visual stimuli with a delayed spike in intracellular calcium [41]. Photostimulation of astrocytes enhanced both excitatory and inhibitory synaptic transmission; this was mediated by metabotropic glutamate receptor (mGluR) activation [42]. Electrical stimulation of the nucleus basalis paired with visual stimulation-induced potentiation of visual responses in neurons found in the primary visual cortex [43]. The mechanism behind these changes was thought to involve muscarinic ACh receptors evoking N-methyl-D-aspartate (NMDA) receptor-mediated slow inward currents (SICs) in neurons. This potentiation was absent, however, in mice lacking the primarily astrocytic IP3 receptor (type 2), suggesting that intracellular calcium elevations in astrocytes were required [43]. Similar to what was seen in the visual cortex, odor stimulation in mice led to calcium transients in astrocytic endfeet and was highly correlated with the dilation of upstream arterioles and the release of glutamate [44]. In addition, odor-evoked functional hyperemia in glomerular capillaries was mediated, in part, by astrocytic mGluR5 and cyclooxygenase (COX) activation.
Activation of the somatosensory cortex through either mechanical limb stimulation [8] or whisker stimulation [7] led to delayed increases in astrocytic calcium in the somatosensory cortex. Whisker stimulation also led to delayed onset local field potential (LFP) responses [45]. In layer 2/3 of the barrel cortex, astrocytic intracellular calcium increases were elicited in response to glutamatergic inputs from layer 4 of the same column, but not to glutamatergic projections from layer 2/3 of adjacent columns [46]. Interestingly, either somatosensory stimulation via tail pinch [47] or whisker stimulation combined with electrical stimulation of the nucleus basalis of Meynert (NBM) [45] induced intracellular calcium spikes in astrocytes and cortical long-term potentiation (LTP). Locomotor performance also led to calcium rises in networks of Bergmann glia, which were abolished by the blockade of either neuronal activity or glutamatergic transmission [6]. Astrocytes in the ventrobasal thalamus preferentially responded to corticothalamic inputs over sensory pathways [48]. Taken together, it is clear that astrocytes respond to a variety of neuronal inputs in a discriminatory manner.
Astrocytes Send Feedback Signals to Neurons
After astrocytes sense synaptic activity, they have the ability to respond with an output signal back to neurons. This has been demonstrated in co-cultures of astrocytes and neurons, where astrocytes were able to trigger calcium increases in adjacent neurons [49,50]. Astrocytes in slices of neonatal rat ventrobasal thalamus displayed spontaneous intracellular calcium oscillations that propagated as waves to neighboring astrocytes and resulted in NMDA receptor-mediated SICs in neurons found along the wave path [51]. In hippocampal slices of rats, direct stimulation of astrocytes increased miniature inhibitory postsynaptic currents (mIPSCs) in pyramidal neurons, an effect that was blocked by inhibition of astrocytic calcium signaling [52]. Similarly, stimulation of either the Schaffer collateral pathway or individual astrocytes resulted in NMDA receptor-mediated SICs in adjacent neurons [53]. Calcium elevations in astrocytic processes have also been proposed to participate in local tuning of neurotransmitter release at excitatory synapses [54].
Release of “Gliotransmitters” from Astrocytes
Chemical signaling is now known to underlie bidirectional communication between astrocytes and neurons (Fig. 3.2) [11]. Gliotransmitters are neuroactive chemicals released from any glial cell, including oligodendrocytes, astrocytes, and microglia. Several compounds may be released from glia, including excitatory amino acids, nucleotides and nucleosides, eicosanoids, prostaglandins, neurotrophins, cytokines [55], and taurine [56]. The release of gliotransmitters, a process known as gliotransmission, primarily occurs in astrocytes. Evidence for gliotransmission began as early as the 1970s [13,57,58], but this concept was not widely accepted. Now, however, there is a growing body of evidence in support of gliotransmission [1,4,12,14,55,59–72].

Neurotransmitters spilling over from active synapses can stimulate receptors on glial cells, resulting in internal calcium elevation and activation of the glial cell (black arrow, synaptic activation of glia). Several different transmitters have been shown to start neuron-to-glia signaling at various synapses of either the central or peripheral nervous system. [Ca2+]i rise in the active glial cell (depicted in pale yellow; pink indicates inactive cells) can produce a neuromodulatory response by triggering transmitter release (blue arrows, neuromodulatory glia). This glia-to-neuron signaling can act locally, feeding back a response to the original stimulatory synaptic domain, or distally, to modulate a different synaptic domain, if the activation signal is spatially propagated in the glial network. Various transmitters may sustain glia-to-neuron signaling, although the best collected evidence so far is for calcium-dependent “exocytosis” of glutamate from astrocytes. A third form of communication, here called “gliotransmission” (red dashed lines and arrows), allows signals to spread in the glial network with spatial expansion of the original input. Mostly studied in cultured astrocytes, gliotransmission has been classically monitored as intercellular waves of calcium elevation (pale yellow cells) and thought to depend on intracellular signal (eg, inositol triphosphate [IP3]) diffusion through gap junctions. But recent work indicates that calcium waves are accompanied by waves of extracellular mediators, such as ATP or glutamate. Therefore, gliotransmission must also proceed through autocrine/paracrine glia-to-glia signaling, possibly involving additional transmitters such as prostaglandins and nitric oxide. Source: Reproduced with permission from Bezzi P, Volterra A. A neuron-glia signalling network in the active brain. Curr Opin Neurobiol 2001;11(3):387–94.
Mechanisms of Gliotransmission
Astrocytes are known to release gliotransmitters through a variety of mechanisms. Early studies used in vitro techniques to demonstrate the ability of astrocytes to release gliotransmitters while more recent studies have observed these phenomena in vivo. The gliotransmitter that has been most well studied is glutamate. At least six potential mechanisms of astrocyte glutamate release have been hypothesized so far: (1) calcium-dependent exocytosis; (2) reversal of membrane transporters; (3) glutamate exchange through the cystine/glutamate antiporter; (4) release through ionotropic purinergic receptors; (5) cell swelling-induced opening of anion channels; and (6) release through hemichannels (Fig. 3.3) [73]. Each of these are discussed in more detail below.

(A) Ca2+-dependent exocytosis; (B) reversal of uptake by plasma membrane glutamate transporters, (C) glutamate exchange via the cystine/glutamate antiporter, (D) release through ionotropic purinergic receptors (only one subunit shown, but exchanger is believed to be multimeric), (E) anion channel opening induced by cell swelling, (F) release through functional unpaired connexins, “hemichannels.” Sb 2, synaptobrevin 2; SNAP-23, synaptosome-associated protein of 23 kDa; Stg 4, synaptotagmin 4; Sx 1, syntaxin 1; V-ATPase, vacuolar type H+-ATPase; VGLUT, vesicular glutamate transporter. Source: Reproduced with permission from Malarkey EB, Parpura V. Mechanisms of glutamate release from astrocytes. Neurochem Int 2008;52(1–2):142–54.
Calcium-Dependent Exocytosis
Several lines of evidence for calcium-dependent exocytosis of glutamate from astrocytes have been accumulating since the 1990s. Initial studies found intracellular calcium rises in astrocytes that were oscillatory and propagating [9]. Next, researchers discovered that astrocytes can release gliotransmitters and that glutamate can induce a response in neighboring neurons [50]. Finally, various vesicular release proteins were discovered to be expressed by astrocytes [74]. Collectively, these data support the notion that astrocytes release glutamate through calcium-dependent exocytosis. Although this mechanism has gained a great deal of support, it still remains controversial [75]. All lines of evidence (and controversy) will be discussed in detail in Chapter 6: Astrocyte Calcium Signaling.
Reversal of Glutamate Transporters
Astrocytes play an important role in removing glutamate from the extracellular space through specific sodium-dependent glutamate transporters found near synapses. The two major astrocytic transporters are glutamate transporter-1 (GLT1) and glutamate/aspartate transporter (GLAST). Under homeostatic conditions, glutamate is driven into astrocytes through its concentration gradient. During pathophysiological events, however, perturbed extracellular ionic concentrations may favor the reversal of these transporters. This was initially demonstrated with whole-cell clamp of Müller cells from salamander retina by Szatkowski et al. [76]. They found that raising extracellular K+ concentrations around glial cells evoked an outward current produced by the reversal of glutamate uptake [76]. This current was inhibited by extracellular glutamate and sodium and was increased by membrane depolarization.
Many studies used primary rat or mouse astrocyte cultures to study gliotransmitter release through glutamate transporter reversal. Massive excitatory amino acid efflux was seen under conditions of energy failure, including blockade of glycolytic and oxidative metabolism and ATP production [77,78]. Prolonged exposure to high extracellular K+ concentrations (60 mM for 15–20 min), however, did not increase extracellular glutamate concentrations [78]. Similar results were seen in pure cultures of retinal neurons and glia preloaded with [3H]-acetate (preferentially metabolized by astrocytes) and [U-14C]-glucose (preferentially metabolized by neurons). After total metabolic blockade with iodoacetate and KCN, release of [3H]-glutamate was significantly increased within 15 minutes whereas [U-14C]-glutamate remained unchanged [79]. Pretreatment with a glutamate transporter blocker inhibited about 57% of the glutamate release after 30 minutes of metabolic block, suggesting that sodium-dependent glutamate transporter reversal is a major contributor, but not the sole component, to increased extracellular glutamate levels during energy deprivation [79]. Similarly, inhibition of sodium-dependent glutamate transport with the astrocytic GLT1 blocker dihydrokainate (DHK) was protective against anoxia-induced glutamate release [80].
Ischemia has been associated with increased extracellular glutamate levels. Forebrain ischemia was induced in anesthetized rats and microdialysate concentrations of glutamate were measured in the presence or absence of various blockers [81]. Inhibition of the astrocytic GLT1 with DHK or anion channel blockers diminished ischemia-induced glutamate release in rat striatum, suggesting that both cell swelling and the reversal of glutamate transporters might contribute to elevated extracellular glutamate levels [81]. A separate study induced ischemia in hippocampal slices from 12-day-old rats and bathed slices with various blockers of different glutamate release mechanisms. They found that reversal of neuronal glutamate transporters was the primary contributor to ischemia-induced glutamate release [82].
Reversal of glutamate transporters has excitotoxic consequences including lipoxygenase pathway-mediated cell death [83]. Exposure of co-cultures of rat cortical neurons and glia to the excitatory amino acid transporter inhibitor L–trans-pyrrolidine-2,4-dicarboxylate (PDC) led to a rapid elevation in extracellular glutamate and NMDA receptor-mediated excitotoxicity [84]. The glutamate increase was insensitive to tetrodotoxin, independent of extracellular calcium levels, present in astrocyte-pure cultures, and was suppressed in sodium-free medium. To confirm these findings, Volterra et al. [84] used glutamate transporters functionally reconstituted in liposomes to show that PDC activated carrier-mediated release of glutamate via transporter reversal. Taken together, these data suggest that the net efflux of glutamate may occur in the unhealthy brain, but the contribution to the healthy brain has yet to be determined.
Cystine/Glutamate Antiporter
Transport of cystine into the cell is essential for the synthesis of glutathione, an important antioxidant. In addition, cystine transport contributes to the maintenance of extracellular basal glutamate levels. Uptake of cystine into cells can occur through the cystine/glutamate antiporter (system xc−) or the sodium-dependent glutamate transporters (system XAG−); both of these are present on astrocytes. Once cystine is driven into the cell, glutamate is transported out. The addition of cystine to astrocytes in vitro or in vivo led to increased extracellular glutamate levels [85–90].
Warr et al. [90] used whole-cell patch-clamp analysis of neurons in rat brain slices to measure glutamate activation of NMDAR receptors after the application of cystine. They found that cystine generated a current, which was abolished by glutamate receptor blockers [90]. This current, however, was not due to the direct activation of NMDA receptors or by potentiation of the action of background levels of glutamate receptors. Moreover, experiments using isolated salamander Müller cells demonstrated that cystine did not block glutamate transporters and cystine-induced currents were not affected by the removal of extracellular chloride or the addition of the (Na+–K+–2Cl−) cotransporter inhibitor furosemide. Their results suggested that the cystine-generated glutamate current was the result of cystine–glutamate exchange in cells [90].
The use of microdialysis has been used to measure glutamate release in response to cystine application in rat brain slices [88] and in vivo [85]. Blocking the cystine/glutamate antiporter in vivo decreased extracellular glutamate levels in the rat striatum by 60% whereas blocking sodium and calcium channels had minimal effects [85]. Similarly, cystine increased tonic glutamate release in CA1 hippocampal slices, measured by glutamate receptor-mediated currents evoked in pyramidal cells [86]. This release was inhibited by the anion exchange inhibitor 4,4ʹ-diisothiocyano-2,2ʹ-stilbenedisulfonic acid (DIDS) but remained unaffected by blocking gap junctions, ATP receptors, specific anion channels, and calcium-dependent release. It is possible, however, that the release of glutamate only occurred with the addition of cystine, but not at physiological levels [86].
The cystine/glutamate transporter may be negatively regulated by mGluR2/3 via a cyclic adenosine monophosphate (cAMP)-dependent protein kinase mechanism [85]. Interestingly, Tang et al. (2003) found that this regulation can occur bidirectionally; low concentrations of mGluR2/3 agonist increased cystine uptake whereas low concentrations decreased cystine uptake [89]. A similar bell-shaped dose response curve was seen with a PKA inhibitor. Blocking calcium/calmodulin-dependent kinase II, however, stimulated system xc−, suggesting it is involved in system xc− modulation [89].
Regulation of synaptic activity through type II mGluRs may be a result of the cystine/glutamate antiporter [87,88]. Specifically, bath application of physiological levels of cystine to acute tissue slices from the nucleus accumbens or prefrontal cortex led to elevated glutamate levels but decreased miniature excitatory postsynaptic currents (mEPSCs), spontaneous EPSC (sEPSC) frequency, and evoked EPSC amplitude [87]. The effects were thought to be presynaptic and were blocked by system xc− or mGluR2/3 antagonists [87]. This mechanism may play a role in drug-seeking behavior because rats who were trained to self-administer cocaine and were also treated with mGluR2/3 blockers resisted the reinstatement of this behavior [87].
The reduction in extracellular glutamate after cocaine withdrawal has been attributed to a compromised system xc− [91]. Both the application of glutamate exchange channel blockers and cocaine diminished extracellular glutamate levels [92]. System xc− was also found to be highly expressed in gliomas and inhibition of system xc− reduced the levels of intracellular glutathione and consequently caused oxidative cell death of tumor cells [93]. In addition, the use of system xc− inhibitors have been used to demonstrate that cystine uptake may be part, but not the sole contributor, to an astrocyte cell death mechanism [83].
Purinergic Receptors
Purinergic signaling may occur through either P1 receptors (ligand is adenosine) or P2 receptors (ligands are ATP, uridine triphosphate (UTP), and their metabolites). P1 receptors are G-protein-coupled whereas P2 receptors can be either GPCRs (P2Y receptors) or ligand-gated ion channels (P2X receptors, Table 3.1). Glial cells express both ionotropic and metabotropic purinergic receptors. Purinergic P2X receptors express several subunits that can assemble into homomeric or heteromeric channels. P2X7 receptors are homomeric, have a large pore, and are found on astrocytes.
Table 3.1
Classification of Purinergic Receptors
Receptor Type | Receptor Name | Main Ligand(s) |
G-protein-coupled receptors (GPCRs) | P1 | Adenosine |
P2Y | ATP, ADP, UTP, UDP | |
Ligand-gated ion channels | P2X | ATP |
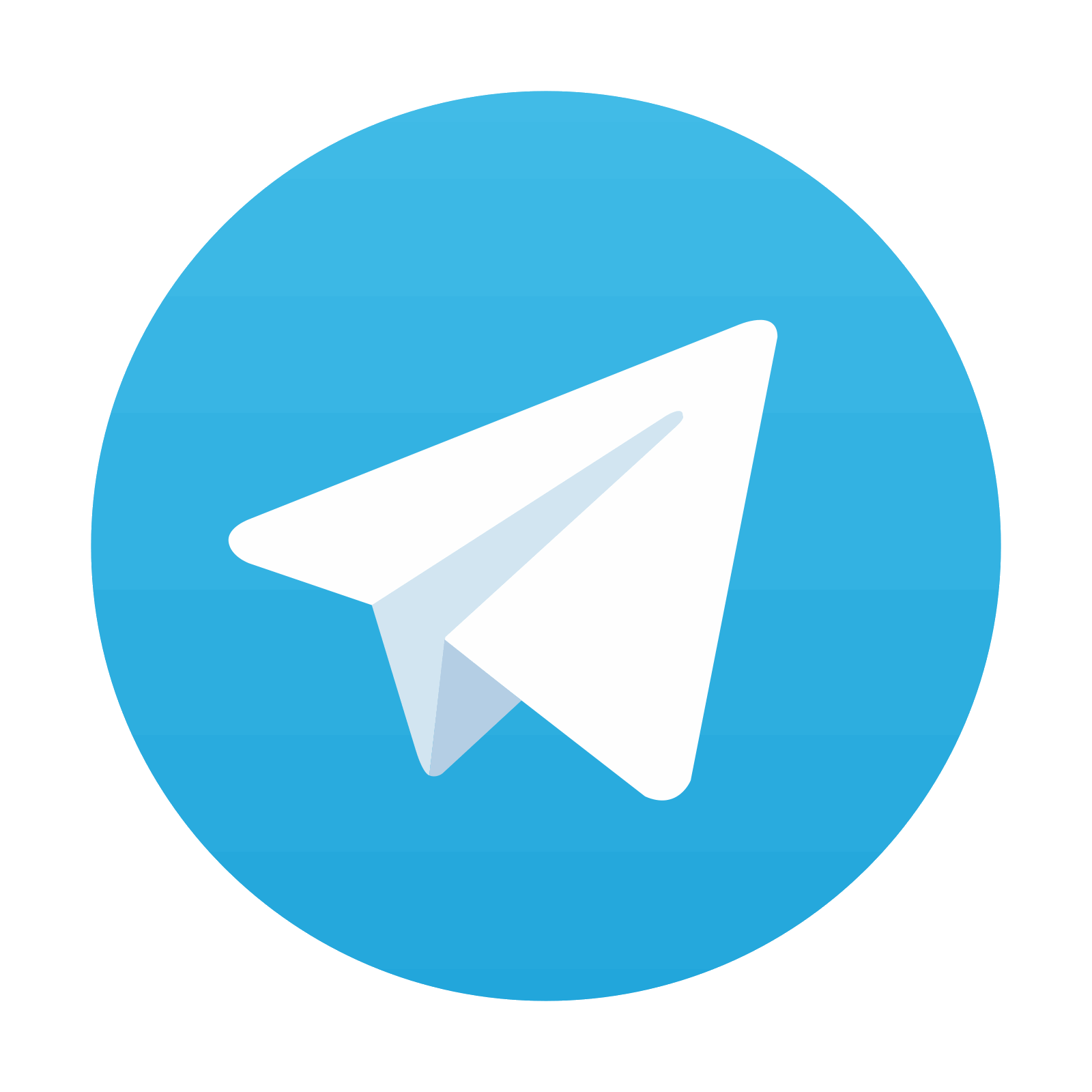
Stay updated, free articles. Join our Telegram channel
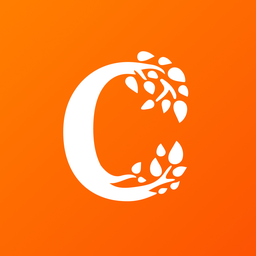
Full access? Get Clinical Tree
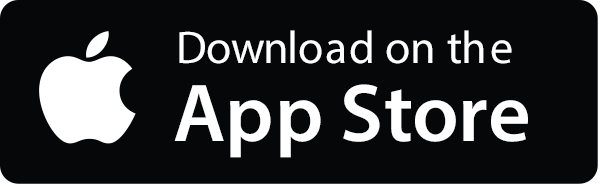
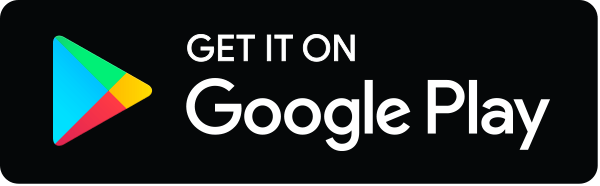
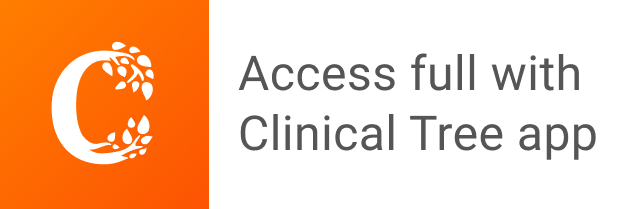