Neuropathology of Human Epilepsy
Abstract
In this chapter, we briefly review salient features of the pathology of human focal epilepsies. We present human histopathological data and classification of hippocampal sclerosis (HS) as well as findings in long-term epilepsy-associated tumors (LEATs) and focal cortical dysplasias (FCDs). We also summarize the pathology of tuberous sclerosis, tumor-associated epilepsy, posttraumatic epilepsy (PTE), and poststroke epilepsy. All of these lesions are associated with intractable focal epilepsy. Detailed histopathologic comparison of epileptic versus normal tissue provides a clearer understanding of the variety of human pathology associated with epilepsy and informs the relevance of specific animal models of epilepsy. It is clear that continued research with human tissue will be critical to continue to define the molecular and cellular changes involved in the large spectrum of human epilepsies.
Keywords
Pathology; hippocampal sclerosis; long-term epilepsy-associated tumors; focal cortical dysplasia; human
Overview
In the 2010 International League Against Epilepsy (ILAE) classification of electroclinical syndromes and other epilepsies, the ILAE report listed epilepsies attributed to structural–metabolic causes [1]. These include:
Malformations of cortical development (hemimegalencephaly, heterotopias, etc.)
Neurocutaneous syndromes (tuberous sclerosis (TS) complex, Sturge-Weber, etc.)
These comprise known epilepsy-related structural causes that are amenable to pathologic evaluation, diagnosis, and classification. In this chapter, we will review some of the features of human epilepsy neuropathology. In particular, we will focus on hippocampal sclerosis (HS), long-term epilepsy-associated tumors (LEAT) and focal cortical dysplasias (FCD). In a large German series of 4512 epilepsy tissue samples, these three pathologies were the most common: HS (40%), LEAT (27%), and FCD (13%) [2]. Therefore, for the purposes of this chapter, we will first review the main features of the neuropathology of HS, LEAT, and FCD; then we will describe possible astrocytic contributions to TS, tumor-associated epilepsy, posttraumatic epilepsy (PTE), and poststroke epilepsy (PSE).
Hippocampal Sclerosis
Temporal lobe Epilepsy and the Development of Epilepsy Surgery
Affecting over 40 million people worldwide [3], temporal lobe epilepsy (TLE) is characterized by recurrent seizure activity originating within the temporal lobe. TLE is the most common form of epilepsy found in adults and seizures are medically intractable in about 40% of patients suffering from this disease [4,5]. Depth electrode studies have shown that most seizures originate from the hippocampus [6]. The most common pathological feature found in these patients is HS, characterized by a pronounced loss of pyramidal neurons, granule cell dispersion, mossy fiber sprouting, astrogliosis, and microvascular proliferation [3,7–10].
The discovery of the importance of HS has paralleled developments in epilepsy surgery and in pathological evaluation.
In case after case we found the cortex to be tough in the anterior and deep portion of the first temporal convolution. This abnormality extended into, and grew more marked in, the uncus and hippocampal gyrus. The tissue was tough, rubbery, and slightly yellow…We have gradually realized the importance of this discovery, as the epileptogenic focus was often shown by electrocorticography to be situated here, and furthermore patients returned with continuing seizure when we had made anterior temporal removals without excision of this area [11].
The earliest resections for epilepsy, from Macewen [12] and Horsley [13] to Krause [14] and Foerster [15], were largely designed to remove cortical areas reflected in patients’ ictal semiology. Following Hans Berger’s discovery of the human electroencephalogram (EEG) in 1929 [16], EEG was adapted to identify and better localize epileptiform abnormalities, in particular in the temporal lobe. In the late 1930s, Wilder Penfield and Herbert Jasper at the Montreal Neurological Institute developed the use of EEG in combination with electrocorticography and functional mapping of “eloquent” brain areas to tailor epilepsy resections [11,17]. The developing concept of “psychomotor” or TLE and its surgical treatment [18–20] led to the advent of anatomically standardized en bloc temporal lobe resection [21–23]. Soon after, it became clear that pure lateral temporal cortical removal was associated with unsatisfactory seizure outcome, and removal of the deep structures including hippocampus and amygdala was required [11,24–28] (see quote above). Bilateral resections were abandoned early since they resulted in dense anterograde amnesia, such as in the famous case of H.M. [29].
Over the last half-century, many modifications to either “tailored” or “anatomic” temporal lobe resections have been adopted. Anatomically standardized resections rely on the concepts that resection of pathology seen on imaging studies will include the epileptogenic zone, and that resection of eloquent areas will be avoided by conforming to certain anatomic boundaries. In contrast, tailored resections emphasize altering degree of resection based on individual pathophysiology, functional mapping of eloquent cortex, and intraoperative electrocorticography [30–33]. Of course, the goal of all epilepsy surgery remains extirpation of the epileptogenic zone without producing neurological or cognitive deficits.
There are several differences among current techniques for TLE surgery. The first is the relative extent of mesial temporal versus neocortical resection, from neocortical resection without mesial resection [34,35] to “anteromedial temporal lobectomy” (limited cortical removal followed by extended hippocampal resection) [36–38] to mesial resection without neocortical resection (selective amygdalohippocampectomy) [39–41]. The second is the exact extent of mesial resection, with variations in amygdalar resection [42,43] and hippocampal and parahippocampal gyrus resection [38,42,44–47]. The third difference is surgical approach (eg, transsylvian [40,41,48] versus transcortical [39,44,49–52] versus subtemporal [53,54] selective amygdalohippocampectomy). A final difference is whether the resection is anatomic or “tailored,” as described above [30–33]. There has been a trend toward reducing the extent of neocortical resection and increasing the extent of resection of mesial structures. Nevertheless, presence of lateral pathology or dual pathology clearly requires either standard temporal lobectomy or tailored approaches.
Patient selection, preoperative work-up [55], anesthesia [56], outcome and complications of TLE surgery will not be considered in detail here. Suffice to say here that the efficacy of TLE surgery is now very well established. Randomized trials have demonstrated clear superiority of surgery versus best medical treatment for TLE [57–59]. Approximately two-third of patients will be seizure-free postoperatively [60], a figure which is remarkably consistent across large-volume epilepsy centers. The interested reader is also referred to other references that present detailed anatomic discussions of the intricate surgical and vascular anatomy of the temporal lobe [37,48,50,61–72].
Development of the Concept of HS
The history of neuropathology of HS provides perspective on the modern concept of HS. In 1825, Bouchet and Cazauvieilh [73] described the consistency of the hippocampus in 18 postmortem evaluations of patients with epilepsy. Induration was noted in four cases, softening in one (also see [74]). However, it was Sommer in 1880 who provided a detailed description of HS in 90 postmortem cases [75]. Sommer found that “all pyramidal cells…which are doubtlessly of greatest importance for the function of Ammon’s horn are missing” [75]. In particular, they were missing in an area which came to be called “Sommer’s sector,” corresponding to the CA1 subfield, which usually exhibits the greatest degree of neuronal loss in HS (Fig. 5.1). Later, Bratz published a series of findings in 70 patients with epilepsy [76] (Fig. 5.2). In addition to confirming and extending the observations of Sommer, Bratz recognized an area that was resistant to degeneration (modern-day CA2). Spielmeyer later reported that in severe cases, all sectors could exhibit degeneration and loss of pyramidal cells (total HS) [77]. In 1966, Margerison and Corsellis reported a postmortem study of 55 epilepsy patients and described another pattern with cell loss and gliosis in the hilus with sparing of Sommer’s sector, termed “endfolium sclerosis” [9]. More recently yet another pattern with more selective CA1 loss has been described [6,10,78–80]. To this day, HS remains one of the most common pathologies seen in patients with medically-intractable focal epilepsy, based on both epilepsy surgery and autopsy data (Fig. 5.3). Obviously, the availability of resected hippocampal tissue from epilepsy surgery has greatly added to the previous postmortem studies. Consistently, HS was present in 30–66% of temporal lobe cases in both early and more recent studies [6,9,75,76,78,81,82]. When present, the sclerotic hippocampus is the predominant focus of seizure origin [83].

Sommer described a vulnerable region of neuronal loss in Ammon’s horn sclerosis that became known as Sommer’s sector (modern-day CA1). In his original paper he describes this as “The whole part of the gray matter that belongs to the medial wall of the lower horn, approximately from the fold-over locus of the ventricular endothelium over the middle of the curvature…or if one considers the Ammon’s horn as an ellipsoid, whose biggest axis goes parallel to the horizontal plane, in a sector in which the lower exterior quadrant (calculated from the medial plane of the brain) covers approximately 60 degrees of the horizontal axis towards the smaller one, and 20 degrees in the upper exterior.” In the figure, these parameters have been superimposed on a surgical resection specimen immunostained with neuronal nuclear antigen to highlight Sommer’s description. Source: Reproduced with permission from Thom M. Hippocampal sclerosis: progress since Sommer. Brain Pathol 2009;19:565–72 (Figure 1).

From an original paper published in 1899 by Bratz depicting the regions of neuronal loss and reduction in volume in the hippocampus compared with a control. Note that the granule cell layer also appears somewhat broader than in the control although the phenomena of granule cell dispersion in the dentate gyrus remained to be described until 1990. Source: Reproduced with permission from Thom M. Hippocampal sclerosis: progress since Sommer. Brain Pathol 2009;19:565–72 (Figure 2).

(A) Luxol-fast blue/cresyl violet-stained preparation of a surgical sample with subfield neuronal loss involving CA1 and CA4. (B) GFAP preparation confirming dense fibrillary gliosis in CA1 with a sharp cut-off point (arrows) with the adjacent subiculum (SBC) and (C) higher magnification detail of the abrupt transition of gliosis at the CA1/subiculum (arrows). (D) Granule cell dispersion as seen with cresyl violet and (E) with NeuN showing clusters of dispersed granule cells (inset shows reelin-positive interneurons and bipolar cells in the molecular layer). (F) Neurofilament-positive neurons with enlarged cell bodies in CA4 of the sclerotic hippocampus. (G) CA1 pyramidal cell layer in a patient with MTLE but no evidence of neuronal loss. (H) CA1 with evidence of partial neuronal loss from mid zone and (I) CA1 with severe neuronal depletion and collapse of the layer. (J) Section of the pes hippocampus from a patient with confirmed classical ILAE type 1 sclerosis in the body; in the pes neuronal loss is limited to the endplate in this case as an illustration of variability in the pattern of atrophy that may occur along the longitudinal hippocampal axis. Bar is equivalent to approximately 1 mm (A, B, J), 250 μm (C, F, G, H, I), and 100 μm (D, E). Source: Reproduced with permission from Thom M. Hippocampal sclerosis in epilepsy: a neuropathology review. Neuropathol Appl Neurobiol 2014;40:520–43 (Figure 1).
HS is related to the broader concept of mesial temporal sclerosis (MTS). The hippocampus often shows the most striking histopathologic changes in TLE, but other associated temporal and limbic areas can be affected as well (hence the term MTS). Sommer and Bratz both described cases of histological damage outside of the hippocampus, such as parahippocampal gyrus and temporal neocortex. Meyer and Beck described amygdala sclerosis [84], which was also recognized later by Margerison and Corsellis [9] and others [85]. Damage to entorhinal cortex and other cortical areas has also been well described [85–88]. Figs. 5.3 and 5.4 demonstrate typical pathological features of HS, including pyramidal cell loss, mossy fiber sprouting (originally described by Sutula [89]), and granule cell dispersion (originally described by Houser [90]).

Mossy fiber sprouting, as described by Sutula in 1989, can be seen in the molecular layer of the dentate gyrus in HS using Timm’s method (A) or dynorphin immunohistochemistry (B). Dispersion of the granule cells of the dentate gyrus in HS is also a common finding and was first described by Houser in 1990 and is illustrated here with neuronal nuclear antigen staining (C). GCL, granule cell layer; IML, inner molecular layer. Source: Reproduced with permission from: Thom M. 2009. Hippocampal sclerosis: progress since Sommer. Brain Pathol 19:565–72 (Figure 4).
Let us now consider astrogliosis and microvascular proliferation, the two remaining hallmarks of HS (Fig. 5.5).

(A) Prominent labeling of voltage-gated potassium channel (Kv1.1) in residual neurons in CA1 in HS; differences were noted in the patterns of expression between hippocampal subfields in HS compared with controls (M. Thom, unpub. obs.). (B) Prominent labeling of cannabinoid receptor is noted in the plexus in the molecular layer of the dentate gyrus in HS. (C) Intense labeling of CA1 neurons with cation-chloride cotransporter (NKCC) in HS. (D) In some cases of HS in epilepsy, particularly in adult onset cases or in the context of recent encephalitis, striking reactive, and “balloon cell” gliosis can be seen in the granule cell layer with a proportion of these cells showing membranous CD34 staining, mimicking a focal cortical dysplasia (inset). (E) Immunolabeling with isoform GFAP-delta highlights prominent numbers of small astroglia, particularly in the subgranular zone, in HS. (F) Immunostaining for gap junction protein connexin 43 (Cx43) demonstrated prominent labeling of astrocytic cells in the subgranular zone and occasionally in the molecular layer in a postmortem HS case. (G) Expression of drug transporter protein, p-glycoprotein, is shown on capillaries within hippocampus. (H) Albumin staining demonstrating focal leakage from small capillary vessels in a case of HS/TLE. (I) Coexpression of vascular endothelial growth factor (VEGF) and hypoxia inducible factor 1 alpha (HIF1-alpha) is shown in pyramidal neurons in HS. Bar is equivalent to approximately 35 μm (A, B, D, E, I); 60 μm (C, E, F, H). Source: Reproduced with permission from Thom M. Hippocampal sclerosis in epilepsy: a neuropathology review. Neuropathol Appl Neurobiol 2014;40:520–43 (Figure 4).
Astrogliosis
One striking hallmark of the sclerotic hippocampus is that while there is a specific pattern of neuronal loss, there is also “reactive gliosis” with hypertrophic glial cells exhibiting prominent GFAP staining and long, thick processes. In contrast with all the studies of hippocampal neuron loss, only a few studies have attempted to quantitate changes in astrocyte numbers and densities in epileptic tissue [91–94]. Previously, it was believed that neuronal loss led to astroglial phagocytosis and, consequently, increased gliosis [5]. This view has changed, however, as reactive changes in astrocytes, or reactive gliosis (astrogliosis), is commonly found in both sclerotic and nonsclerotic hippocampal tissue specimens. Gliosis commonly involves fibrillary gliosis in CA1 and radial gliosis in the dentate gyrus [10]. These glial cell changes have become a hallmark of the sclerotic hippocampus and involve astrocyte hypertrophy [4,91,94–98], increased expression of glial fibrillary acidic protein (GFAP) [4] and vimentin [7,99,100], and changes in glial specific proteins [7,95,100]. Thus, it is becoming increasingly clear that multifaceted changes in astrocyte phenotype may play more of a causative role in the overall pathology and seizure susceptibility [5,95,101–103] (topic of the remainder of this book). A recent report indicates a positive correlation between chronic seizure burden and degree of gliosis, and interestingly an increase in reactive astrocyte number in CA3 was the strongest predictor of poor postoperative seizure outcome [104].
Microvascular Proliferation
Interestingly, both Sommer and Bratz commented on abnormalities of the microvasculature in epileptic specimens. In sclerotic areas the vessels were “thick walled and glossy” [76]. Abnormalities of microvasculature, however, were subsequently mostly overlooked until recently. It is now known that there is proliferation of microvessels, increased vascular endothelial growth factor (VEGF) expression, and loss of blood–brain barrier (BBB) integrity in HS [105,106]. Vascular leakage of proteins such as albumin or IgG may also promote neuronal dysfunction or contribute to epileptogenesis [107,108]. Indeed, it has been shown that focal opening of the BBB can promote epileptogenesis, not only in HS but in other focal epilepsies [105,106,109]. In addition, in HS, overexpression of drug transporter proteins such as P-glycoprotein at the BBB facilitate drug efflux, contributing to treatment failure [110,111].
Recently, the Neuropathology Task Force of the ILAE has issued a reclassification of HS. The aim was to synthesize previous attempts to classify the various patterns of hippocampal neuronal loss and to correlate subtypes with postsurgical outcomes. Ultimately, they reached consensus on the following classification [112]:
ILAE type 1: Severe neuronal loss and gliosis predominantly in CA1 and CA4 regions;
ILAE type 2: Predominant neuronal cell loss and gliosis in CA1;
ILAE type 3: Predominant neuronal cell loss and gliosis in CA4;
No HS: Normal content of neurons with reactive gliosis only.
HS ILAE type 1 is more often associated with injury before age 5, early seizure onset, and favorable postoperative seizure control. ILAE types 2 and 3 are possibly associated with poorer postoperative seizure control [112]. Given the heterogeneity of HS pathology (Fig. 5.6) [10], further clinical studies will be needed based on the new ILAE classification to stratify epidemiologic factors, imaging data, and surgical outcome data.

(A) Surgical hippocampectomy specimens, which on histological examination correlated to (Ai) endfolium gliosis with no evidence of sclerosis; (Aii) ILAE Type 3 HS (endfolium sclerosis); (Aiii) ILAE type 2 HS (CA1 predominant sclerosis); and (Aiv) ILAE type 1 HS (classical hippocampal sclerosis). In all of the images, the arrow indicates the pyramidal cell layer of CA1. (B) A 9.4 T MRI image of a postmortem hippocampus from a patient with longstanding epilepsy and ILAE type 2 (CA1 predominant pattern) of HS at this level (shown in (C) in a Luxol-fast blue/cresyl violet preparation), although in other levels the pattern was type 1 (classical). The MRI has the ability to identify subfields and white matter tracts and, indistinctly (arrowed), the dentate gyrus. Improved high-fields sequences in the future may be able to identify and define patterns of HS preoperatively. The MRI image was provided with courtesy of Dr Sofia Eriksson at the Department of Clinical and Experimental Epilepsy, UCL, Institute of Neurology. (D–I) Paired sections of hippocampus from one hemisphere labeled with (D–F) GFAP/counterstained with cresyl violet and (G–I) calretinin: at the level of the subthalamic nucleus (STNc; D, G), lateral geniculate nucleus (LGNc; E, H), and hippocampal tail (F, I). Classical pattern (ILAE type 1) HS is seen in the anterior levels with sprouting of calretinin-positive fibers visible in the dentate gyrus (arrow) at this low magnification; in the tail gliosis and neuronal loss is visible in the CA4 region of the hippocampal tail. Bar is equivalent to approximately 3 mm (D–I). Source: Reproduced with permission from Thom M. Hippocampal sclerosis in epilepsy: a neuropathology review. Neuropathol Appl Neurobiol 2014;40:520–43 (Figure 5).
Tumor-Associated Epilepsy and LEATs
Tumor-Associated Epilepsy
Tumor-associated epilepsy occurs in 20–45% of patients with primary brain tumors (PBTs) [113–115]. These seizures typically manifest as focal seizures with or without secondary generalization and are commonly refractory to antiepileptic drug (AED) treatment. Surgical removal of tumors usually results in seizure control, but many tumors cannot safely be resected. The underlying pathophysiology of seizures secondary to brain tumors is poorly understood. However, a variety of hypotheses have been proposed including altered neuronal regulation and connections, deranged vascular permeability, abnormal BBB, and impaired glial cell function. The tumor itself may be the seizure focus or the tumor may cause secondary perilesional tissue alterations such as growth, inflammation, edema, or necrosis triggering seizure activity.
Tumor histology influences the propensity to generate seizures. Low-grade PBT grow slowly and invade normal surrounding tissue and have a high frequency of epilepsy. For example, dysembryoblastic neuroepithelial tumors (DNTs) and Grade II astrocytomas exhibit this kind of activity [116,117]. Glioneuronal malformations such as gangliogliomas and FCDs lack normal neuronal organization as well as connections and can form epileptic foci [118]. High-grade gliomas such as glioblastoma multiforme (GBM) exhibit a lower frequency of seizures [116,117]. The seizures in these lesions may originate in areas of necrosis and hemosiderin deposition [119].
Tumor location also plays a critical role. Both intra-axial as well as extra-axial PBTs may be associated with seizures. Hence, a variety of mechanisms may contribute to seizure generation, as extra-axial tumors compress normal brain tissue, whereas intra-axial tumors infiltrate normal brain tissue. Intra-axial location may also influence epileptogenicity, with temporal or frontal lobe location being more associated with seizures than other lobes [113,120–122]. Infratentorial as well as sellar tumors seldom cause seizures [113].
Alterations in the peritumoral brain microenvironment may predispose to seizure generation. Some of the common changes seen in PBT are altered BBB function, enzymatic changes, and impaired intercellular connections. BBB alteration or disruption may lead to abnormal leakiness and extravasation of plasma proteins or other substances which may lead to increased excitability and seizures. For example, it is known that focal disruption of the BBB can lead to the development of a seizure focus [123]. The peritumoral microenvironment is also associated with altered levels of enzymes such as lactate dehydrogenase, cAMP phosphodiesterase, enolase, and thymidine kinase which may cause metabolic imbalance [124]. Intercellular connections between adjacent glial cells occur via connexin transmembrane gap junction proteins [125]. Altered expression of connexins has been found in epilepsy-associated brain tumors [125] which may also predispose the perilesional epileptic cortex to hyperexcitability.
Other alterations in the peritumoral microenvironment may contribute to seizure generation. PBT have a relatively higher metabolic rate than normal brain tissue. This creates a relative hypoxia and interstitial acidosis. Marked vasogenic brain edema is commonly seen around brain tumors, and increased tissue water content may also modulate neural excitability [126]. In addition to increased water content, an increase in sodium, calcium, and serum proteins have been seen in quantitative studies of peritumoral tissue [127].
Glutamate, the major excitatory neurotransmitter in the brain, may contribute to perilesional seizure generation. A “glutamate hypothesis” of tumor-associated epilepsy has been advanced which suggests that tumors excite surrounding tissue by glutamate overstimulation. Several lines of evidence are relevant to this hypothesis. (1) Microdialysis studies of gliomas have revealed reduced glutamate in the tumor compared to peritumoral tissue, consistent with glutamate release from the tumor [128]. (2) Changes in ionotropic and metabotropic glutamate receptors (mGluRs) may contribute. Gliomas exhibit a high concentration of ionotropic glutamate receptors (AMPA, NMDA and kainate receptors). The glutamate receptor subunit GluR2 has been found to be underedited at the Q/R site in gliomas, which would increase AMPA receptor Ca2+ permeability and potentially result in increased glutamate release by glioma cells [129]. Alternatively, sustained NMDA receptor activation may represent a pathological mechanism for seizure generation [130]. Astrocytes in perilesional areas demonstrate increased expression of kainate receptors [131]. Activation of kainate receptors has been demonstrated to downregulate inhibitory stimuli which may predispose to formation of an epileptic focus in the perilesional area [132]. Metabotropic glutamate receptors (mGluR) lead to intracellular signaling through guanosine triphosphate–related (GTP) proteins and protein kinase cascades resulting in long-term neuromodulation [131]. Multiple subtypes of mGluRs have been found to be overexpressed in reactive astrocytes in the perilesional zone around tumors compared to normal cortex [131]. (3) Sontheimer’s group found that glioma cells release larger than normal amounts of glutamate in vitro [133]. The release of glutamate from glioma cells was accompanied by a marked deficit in Na+-dependent glutamate uptake, reduced expression of astrocytic glutamate transporters, and upregulation of cystine–glutamate exchange [134]. Hence, glioma cell release glutamate at the margins of the tumor may initiate seizures in peritumoral neurons.
Glutamate may also be involved in tumor invasion. One recent hypothesis of tumor invasion proposes that glioma cells use release of glutamate to induce excitotoxic destruction of neurons to create new micro-passages for migration of tumor cells [134–137]. Neoplastic cells have inherently higher glutamate concentrations that serve as an autocrine trigger to increase rhythmic oscillations of calcium usually necessary for cellular migration [138]. These intrinsically higher glutamate concentrations may also lead to local hyperexcitability along the paths of glioma cell migration.
Alterations in GABA, the main inhibitory neurotransmitter in the brain, may also contribute to tumor-associated seizures. It would be simplistic to assume that increased GABA activity would always suppress epileptic activity. GABA does not always hyperpolarize neurons; based on the GABA reversal potential (itself determined by expression of chloride transporters), GABA can depolarize neurons [139–141]. Studies of PBTs demonstrate increased GABA immunoreactivity [142,143], but further studies will be necessary to determine the exact role of the GABA system in contributing to the hyperexcitability of perilesional areas. Recent data from the Sontheimer laboratory in a validated mouse glioma model demonstrated reduction in peritumoral parvalbumin-positive GABAergic inhibitory interneurons (which would disinhibit the peritumoral neuronal networks) [144].
A final distinct potential mechanism underlying tumor-associated epilepsy is altered K+ homeostasis. In support of this hypothesis, both reduced Kir currents [145] and mislocalization of Kir4.1 channels [146] have been found in malignant astrocytes.
Long-Term Epilepsy-Associated Tumors
Any brain tumor can generate seizures, but the term “long-term epilepsy-associated tumors” (LEAT) has been applied to various lesions found in patients with long histories (>2 years) of medically-intractable epilepsy [147–149]. LEATs differ from most other PBTs in: young age of onset, slow growth, presence of some neuronal (in addition to glial) differentiation within the tumor, and neocortical location (often temporal lobe) [148]. The most common LEATs are gangliogliomas and dysembryoplastic neuroepithelial tumors (DNTs). Gangliogliomas represent the most frequent tumor in patients with focal epilepsy [150–153]. These lesions contain both a highly-differentiated glial component and also a dysplastic neuronal component (Fig. 5.7). DNTs, by contrast, are composed of floating neurons and oligodendroglia-like elements [154,155]. These lesions are on a continuum with types of FCDs associated with epilepsy [156], the main distinction being that FCDs have no proliferative component [153]. In the 2011 ILAE FCD classification [157], tumor-associated cytopathological changes are categorized as type IIIb (see below and Table 5.1).

(A) Aggregates of atypical ganglion cells of varying size typically with neurofilament positivity demonstrated (inset) are diagnostic criteria. (B) Clusters of CD34 positivity cells around dysplastic neurons are often identified with scattered intermingled astrocytic cells (inset). (C) and (D) LEAT with features of diffuse ganglion cell tumor in the temporal lobe with cortex on right side comprising diffuse and subcortical nodular aggregates (arrowhead in C) of neuronal islands with atypical neurons (inset in C) within islands but no glial component (Luxol-fast blue/cresyl violet preparation). In the same case (D), neurofilament highlights normal orientation of cortical neurons (arrowhead) as well as the single dysmorphic white matter neurons (arrow in main picture and top inset); CD34 was focally expressed around abnormal neurons (bottom inset). (E) A mixed glioneuronal tumor with rarefaction and pallor of the white matter beneath a diffusely infiltrated cortex (arrowhead) but without cavitation or cystic change. (F–H) Cortex adjacent to a ganglioglioma. (F). H&E shows a disrupted cortical architecture which may mimic a cortical dysplasia. (G) NeuN reveals the vestiges of an overrun cortex with residual lamination, including layers II and IV, recognizable. (H) CD34 staining reveals scattered multipolar cells, including in layer I. Scale bar (A), (B)=40 mm; (C)=100 mm; (D–H)=115 mm. H&E=hematoxylin and eosin; LEAT=long-term epilepsy-associated tumor; NeuN=neuronal nuclear antigen. Source: Reproduced with permission from Thom M. et al. Long-term epilepsy-associated tumors. Brain Pathol 2012;22:350–79 (Figure 1).
Table 5.1
ILAE Classification of Focal Cortical Dysplasias (FCD)
FCD Type I (isolated) | Focal Cortical Dysplasia with abnormal radial cortical lamination (FCD Ia) | Focal Cortical Dysplasia with abnormal tangential cortical lamination (FCD Ib) | Focal Cortical Dysplasia with abnormal radial and tangential cortical lamination (FCD Ic) | |
FCD Type II (isolated) | Focal Cortical Dysplasia with dysmorphic neurons (FCD IIa) | Focal Cortical Dysplasia with dysmorphic neurons and balloon cells (FCD IIb) | ||
FCD Type III (associated with principal lesion) | Cortical lamination abnormalities in the temporal lobe associated with hippocampal sclerosis (FCD IIIa) | Cortical lamination abnormalities adjacent to a glial or glioneuronal tumor (FCD IIIb) | Cortical lamination abnormalities adjacent to vascular malformation (FCD IIIc) | Cortical lamination abnormalities adjacent to any other lesion acquired during early life, for example, trauma, ischemic injury, encephalitis (FCD IIId) |
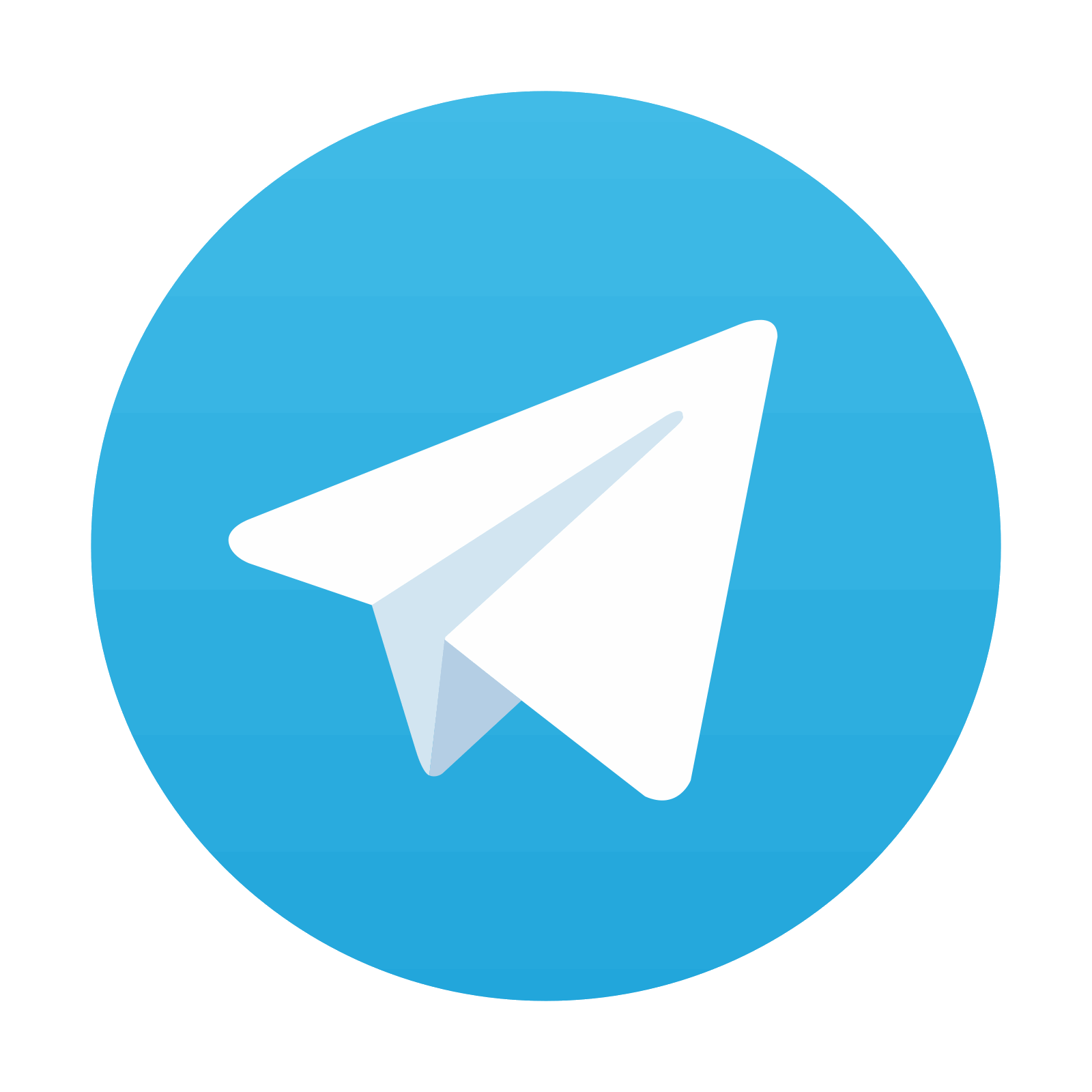
Stay updated, free articles. Join our Telegram channel
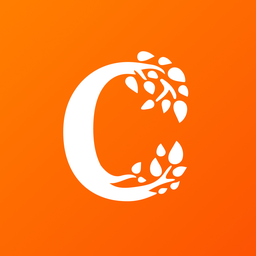
Full access? Get Clinical Tree
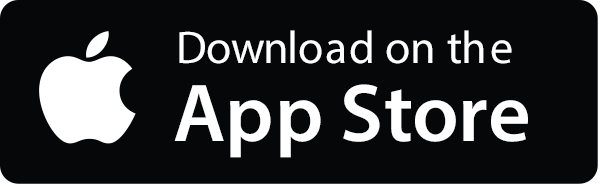
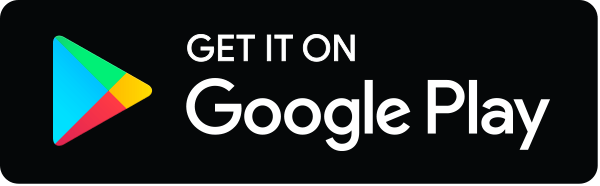
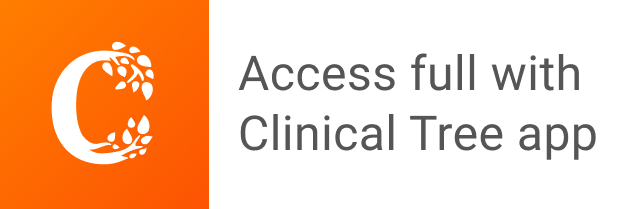