Introduction
Mammals have evolved sophisticated thermoregulatory systems to maintain body temperature within an optimal range for cellular biochemical reactions. Under normal conditions, excluding hibernating mammals, core temperature is maintained around 37° C. However, body temperature fluctuates over the day with an early morning nadir and peak in the evening and with activity. Temperature also is lower in older individuals and males and will vary according to the site at which it is measured. Mean rectal temperature is between 36.7° C and 37.5° C, whereas mean axillary temperatures are between 35.5° C and 37° C. Minor fluctuations around the set-point of 37.5° C are well tolerated, but variation that exceeds a few degrees may be deleterious. Body temperature results from a balance between mechanisms for heat production and mechanisms for heat dispersion. These mechanisms are coordinated by the central nervous system, specifically, by the preoptic and anterior hypothalamic nuclei. The brain itself is particularly sensitive to temperature. In general, hyperthermia is associated with exacerbation of brain injury, whereas induced hypothermia, or even prevention of fever may be neuroprotective. Brain temperature (BT) may therefore be an important therapeutic target; however, in clinical practice, core body temperature is commonly used as a surrogate.
This chapter reviews the physiology of brain temperature regulation, literature regarding the clinical impact of temperature abnormalities on brain injury, and methods to monitor brain temperature. In addition, methods to measure body temperature are briefly reviewed.
Brain Temperature Under Physiologic Conditions
The Brain as a Heater
All metabolic processes produce heat. For example, glucose and oxygen are converted via the Krebs cycle into adenosine triphosphate (ATP), water, and heat. The brain consumes 25% of total body glucose and 20% of total body oxygen for ATP production. About 43% of the energy contained in glucose is converted to ATP, and 67% is dissipated as heat. Given its high metabolic activity, the brain generates a considerable amount of heat.
Maintaining a constant brain temperature depends on a “tight” balance between heat production and heat dissipation. The brain dissipates heat primarily to the systemic circulation. Blood entering the brain is at core temperature, which is lower than brain temperature. Heat is transferred from the brain to the circulating blood. Increased cerebral blood flow (CBF) increases heat transfer and therefore brain cooling. As a result of this heat transfer, the temperature of venous blood leaving the brain in the internal jugular vein is greater than core blood temperature. On average, the gradient between brain and body core temperature is modest, ranging from 0.3° C to 1.1° C, and usually is higher in the brain. There is a reasonable correlation between BT and systemic measures of temperature; however, this relationship changes at the extremes of the physiologic temperature range such that the brain-body gradient may be reversed with brain hypothermia or hyperthermia. Some consider a reversal of this gradient a poor prognostic sign in traumatic brain injury (TBI).
In some species, anatomic variations in brain vasculature improve cooling efficiency. In dogs, goats, and sheep the internal carotid artery may be small or absent, and blood circulates to the skull base via the external carotid artery. Before entering the circle of Willis, the external carotid artery divides into a series of small arteries, “the carotid rete,” or “rete mirabilis,” which flow through the cavernous sinus. During panting, evaporation in the oral and nasal cavities lowers venous blood temperature in the cavernous sinus. Heat then is transferred from arterial blood entering the brain in the carotid rete to venous blood in the cavernous sinus, thus facilitating heat removal from the brain.
Heat also may be dispersed outward by direct conduction through the calvaria, but the skull acts as an insulator, impeding efficient heat exchange. However, when the skull is opened, for example, after decompressive craniectomy, a temperature gradient forms between exposed (noninsulated) brain and ambient temperature; this may result in a BT that is lower than core temperature.
Brain Temperature Is Heterogeneous
There is spatial variation in brain temperature. Structures that are more metabolically active or deeper have higher temperatures. A thermal gradient exists within the brain that allows heat transfer from deep gray structures to white matter to cortex. This has been confirmed in human volunteers by proton magnetic resonance spectroscopy. Furthermore, when temperature probes are withdrawn from the lateral ventricle to the brain surface, slight (nonstatistically significant) temperature differences are detected. In patients with hydrocephalus, BT has been measured at various depths beneath the pial surface. Temperature increases gradually as a function of depth, and the highest temperature is measured in the ventricle.
Brain Temperature Is Dynamic
Because the determinants of brain temperature are dynamic, brain temperature is dynamic. Heat production is determined by cerebral metabolic rate, and heat removal depends on regional CBF and arterial blood temperature. Each of these variables fluctuates. The first BT measurements were obtained in animals in which large BT fluctuations—up to 2° C to 3° C —were observed on exposure to various environmental challenges and upon performance of different behaviors and tasks.
When systemic temperature increases abruptly, e.g., in the heat shock, high fever, or strenuous physical exercise, systemic arterial temperature may increase to a point where the normal temperature gradient between blood and brain disappears or is even reversed. Conversely, BT may decrease below core temperature when brain metabolism is inhibited, for example, profound general anesthesia. Another “special case” is the intentional induction of systemic hypothermia, for example, after cardiac arrest. External or internal cooling reduces core and blood temperature, leading to a sharp gradient between the hot brain and the cooled blood. Specific studies documenting the effect of systemic hypothermia on BT, such as after cardiac arrest, are limited only to a few cases. Variations in metabolic rate or in CBF in the single patient can change or reverse the gradient between BT and core temperature. This may in part explain variable findings on the gradients between core and brain temperature reported in the literature.
Clinical Relevance of Brain Temperature
Brain Temperature and Intracranial Pressure
Because the cellular machinery for energy production is sensitive to temperature, changes in BT may have profound consequences. In particular BT, brain metabolic rate, oxygen, and glucose consumption are linked. Furthermore temperature elevations have been reported to increase inflammatory processes, neuronal excitotoxicity, neurotransmitter release, free radical production, and intracellular glutamate concentrations, and potentiate the sensitivity of neurons to excitotoxic injury in the brain. These may influence cerebral metabolic rate of oxygen (CMRO 2 ) that is physiologically coupled to CBF through modulation of vessel diameter. Therefore an increase CMRO 2 results in vasodilation and increased CBF, which in turn, leads to increased cerebral blood volume. This increase in blood volume is compensated by egress of cerebrospinal fluid from the cranial vault and collapse of low-pressure cerebral veins. Once these compensatory mechanisms are exhausted, intracranial pressure (ICP) increases. Clinically, an increase in ICP may be observed with fever in patients with acute brain damage. This ICP increase may be a surrogate for increased fuel delivery to the brain in the setting of increased metabolic demand. It has been demonstrated that during fever there may be preservation of a normal cerebral lactate/pyruvate ratio (measured by microdialysis) indicating adequate substrate delivery. However, once maximal cerebral vasodilation occurs, further increases in CMRO 2 may not be compensated by further increases in CBF and there may be cellular energy crisis. On the other hand microdialysis studies show that induced normothermia can attenuate the fever-associated metabolic distress.
The relationship between BT and ICP is not simple. A clear relationship does not exist when cumulative BT and ICP data are pooled. In a population, ICP depends on several factors and cannot be predicted solely by BT. However, in certain individual cases an association between ICP and BT may be detected.
Hyperthermia Is Deleterious in Experimental Models of Brain Injury
In the experimental setting it has been demonstrated that hyperthermia aggravates neuronal injury after traumatic or ischemic insults to the brain. Even mild hyperthermia may exacerbate outcome. In animal models of focal cerebral ischemia, infarct volume varies with temperature and any deleterious effect is proportional to the degree of hyperthermia. The impact of hyperthermia (and also hypothermia) on intracerebral hemorrage (ICH) is less clear. In an ICH model in rats, mild to moderate temperature elevations do not worsen outcome, although induced hypothermia can improve outcome. Hyperthermia may damage uninjured brain cells; cell membranes and mitochondria are selectively vulnerable. Damage is not limited to neurons but also involves glia and endothelial cells. Consequences of hyperthermia include increased glutamate release, disruption of the blood-brain barrier, increased proinflammatory cytokines and exacerbation of inflammatory cascades, up-regulation of various enzymes, and expression of heat shock proteins, among others.
Fever Is Associated with Worse Outcomes After Severe Brain Injury
Fever is common after severe brain injury. However, the precise mechanism by which neurologic injury results in fever is still to be fully elucidated. This may include direct injury to the thermoregulatory centers in the hypothalamus. In addition, fever is common when there is intracranial hemorrhage and, in particular, intraventricular hemorrhage. Other risk factors include the severity of injury, depressed consciousness, and use of antiepileptic drugs, especially phenytoin. Although a causal role has not been established in humans, an association between hyperthermia and worse outcome has been demonstrated in patients admitted to neurocritical care for various pathologies. For example, observational clinical studies demonstrate that elevated body temperature is associated in a dose-dependent way with longer intensive care unit (ICU) and hospital length of stay, higher mortality, and worse hospital disposition. An association between fever burden and worse outcome has been found in subarachnoid hemorrhage, and in acute ischemic stroke there is a strong relationship between admission temperature and mortality. Higher temperature also is associated with an increased risk of hemorrhagic transformation of an ischemic stroke. In patients with ICH who survive the first 72 hours after hospital admission, duration of fever is associated with poor outcome, and fever seems to be an independent prognostic factor in these patients. Fever also is common after TBI, especially in the more severe cases. In a meta-analysis of 39 studies that included more than 14,000 patients Greer et al. found a consistent association between higher body temperature and worse outcomes across multiple outcome measures.
Some recreational drugs cause metabolic neural activation and often increase oxygen consumption and heat production. This is typical of cocaine, heroin, amphetamine-like substances, and ketamine. Brain temperature has been studied in rats during cocaine administration, which confirms a dose-dependent temperature increase. Heat stroke causes hyperthermia without an elevation in the hypothalamic set-point. It is characterized by a core body temperature greater than 40° C due to exposure and is accompanied by hot and dry skin, nervous system failure (including delirium, convulsions, and coma), and a systemic inflammatory response. It may culminate in multiorgan system failure and death.
Therapeutic Hypothermia
The neuroprotective effects of hypothermia have been demonstrated in the experimental setting in models of ischemia and trauma. In the clinical setting therapeutic hypothermia is associated with decreased mortality and improved neurologic outcome after cardiac arrest. There also may be a benefit to prehospital hypothermia for cardiac arrest. Consequently the European Resuscitation Council and American Heart Association guidelines recommend the use of hypothermia in patients after cardiac arrest. Hypothermia also is thought to be associated with better neurologic outcome after neonatal birth asphyxia. Prophylactic hypothermia as a method for neuroprotection after TBI in humans has received considerable interest. Multiple single-center studies have suggested benefit; however, two large multicenter trials failed to show efficacy. A meta-analysis suggested that prophylactic hypothermia was associated with a nonsignificant reduction in mortality and a significant improvement in functional outcome. Virtually every single center study has suggested that hypothermia can help control intracranial hypertension after TBI. There is a consensus, however, that hypothermia is not necessary if ICP is controlled with other therapies. Two multicenter randomized trials to examine hypothermia in TBI are in progress in Europe (Eurotherm; www.eurotherm3235trial.eu/home/index.phtml ) and in Australia and New Zealand (POLAR; www.anzicrc.monash.org/polar-rct.html ). In addition, it does not appear that routine pharmacologic or physical cooling provides a benefit in stroke patients. One promising new area for induced hypothermia (core temperature of 32° C to 34° C) is for patients with acute liver failure and encephalopathy.
Most studies of therapeutic hypothermia have measured core body temperature, and data on BT during hypothermia are scarce. Nordmark et al. recorded brain temperature from four patients undergoing therapeutic hypothermia (32° C to 34° C) after cardiac arrest and observed brain temperatures as low as 32° C. There was a decrease in the lactate/pyruvate ratio that paralleled induction of hypothermia. Yoo et al. compared brain and axillary temperature in 54 patients submitted to normothermia and 9 to hypothermia: brain temperature was lowered below normal but remained consistently higher than axillary temperature by approximately 1° C.
Fever Control and Induced Normothermia
Fever in the neurocritical care unit (NCCU) is very common and its adverse effect on brain injury is well described. Hence fever control rather than induced hypothermia may be a reasonable treatment option in the NCCU. Consistent with this premise it appears that hypothermia does not provide any added benefit to cerebral protection compared with normothermia among patients undergoing brain surgery. Clinical studies show that prophylactic normothermia using intravascular cooling can reduce significant increases in BT in patients with severe TBI and in a 2010 case control study, Badjatia et al. observed that fever control may be associated with improved outcome after subarachnoid hemorrhage (SAH). Several recent advances in temperature management mean that fever control and maintaining normothermia now are feasible on a consistent basis in the NCCU. However, there are still many unanswered questions about its use, such as in which patients, indications, and when to start fever control.
Brain Temperature Monitoring
Systemic and Core Temperature
Systemic or core temperature is routinely measured in every patient in the NCCU. From this, brain temperature is inferred. However, several lines of evidence show that brain temperature differs from core temperature and cannot be estimated reliably by monitors placed outside the brain, including the tympanic membrane.
It is rare that temperature is monitored using a thermometer. Instead, thermocouple or thermistor devices, infrared sensors, or liquid crystal devices are used. Thermocouples are based on the phenomenon that a conductor subject to a temperature gradient will generate a voltage that can be measured. A thermistor is a semiconductor that varies its resistance according to temperature. Infrared sensors (e.g., tympanic membrane thermometers) detect heat energy given off by radiation; that is, they do not need to be in direct contact with the object measured. However, this may affect accuracy because the device picks up temperature from the external ear rather than the tympanic membrane. Newer tympanic membrane thermometer models are more accurate. Thermotropic liquid crystals usually are incorporated into disposable plastic strips and have a range between 34° C and 40° C. They are not as accurate as other monitors.
There are several sites from which temperature can be monitored. Broadly speaking the sites can be divided into: (1) core or central sites that represent the temperature of highly perfused organs, for example, esophageal, pulmonary artery, nasopharynx, or tympanic membrane, the last because of its proximity to the internal carotid artery; (2) intermediate sites (e.g., oral [sublingual], rectal, or bladder); or (3) peripheral (e.g., forehead skin or axilla). Core or central sites are preferable because they are less influenced by vasomotor or thermoregulatory mechanisms. Intermediate sites tend to lag behind central monitors in a response to temperature changes and may be influenced by urine flow (bladder) or bacteria in rectum (rectal), whereas peripheral sensors are affected by many environmental influences.
Direct Brain Temperature Monitoring Methods
Brain temperature can be measured directly using probes placed into cerebral tissue or the lateral ventricles. The first thermal recording from the brain of an awake animal dates back to 1860, when the relationship between temperature and brain activation was noted. Intraventricular monitors provide a global (average) measure of brain temperature, whereas intraparenchymal temperature monitors (which are often coupled with brain tissue oxygen sensors) measure regional temperature. Various commercially available probes exist for clinical use ( http://raumedic.com ; www.integra-ls.com ). An earlier version of the Licox (Integra Lifesciences, New Brunswick, NJ) system included separated sensors for brain oxygen and brain temperature. Newer Licox probes incorporate both sensors in a single probe. In vitro analysis of the new Licox probe for simultaneous reading of brain oxygen tension and BT reveals a tendency to underestimate BT, measuring a mean of 0.67 plus or minus 0.22° C below the reference experimental temperature. There is ongoing work to be able to incorporate BT into a single probe that can monitor multiple parameters.
Brain temperature also has been studied noninvasively using magnetic resonance spectroscopy (MRS) and diffusion magnetic resonance imaging (MRI). Focal readings, in volumes as small as 4 cm 3 , have been performed with limited accuracy (approximately 1° C). In addition, BT is higher than that of the extracranial structures with wide variations observed depending on the regions studied. In contrast, Childs et al. found that the average BT was 0.5° C lower than temperature measured by MRS at three extracerebral sites, including oral cavity, tympanum, and temporal artery. In MR-based studies the mean brain-body (rectal) temperature difference is about 1.3 plus or minus 0.4° C.
Brain Temperature Monitoring Complications
Complications associated with the Licox monitor including the BT probe are very rare and generally are associated with insertion of the bolt to hold the probes rather than the monitor itself. Similar to other invasive intracranial monitors, the main risks include cerebral hemorrhage and infection.
Brain Temperature and Brain Oxygen Tension
Temperature has an effect on brain tissue oxygen tension. Given a constant tissue oxygen content, pressure is proportional to temperature, according to the fundamental gas laws. Therefore oxygen tension increases during hyperthermia and decreases during hypothermia because of this biophysical property. Hypoxia itself may induce hypothermia. For this reason the Licox probe used to measure brain oxygen includes a temperature correction tool in its monitor, whereas the new Licox probe incorporates BT in its measurements. Oxygen tension may vary with changes of BT for several reasons, such as CBF changes, shift of the oxyhemoglobin curve, and possible changes in metabolic rate, among others. Interpretation of brain oxygen tension during hyperthermia or hypothermia therefore requires consideration of these factors. Similar changes in oxygen associated with temperature are observed in normal individuals, for example, during exercise.
Brain Temperature and Local Cerebral Blood Flow Measurement
BT can be used to determine local CBF (see Chapter 31 ). This CBF monitor (Hemedex) is based on the principle that thermal conductivity of cerebral cortical tissues varies proportionally with CBF. Therefore measurement of thermal diffusion at the cortical surface may be used to determine CBF. This monitoring system consists of two small metal plates (thermistors) that are inserted into brain tissue. One of the plates is heated, thereby establishing a temperature gradient between the two thermistors; this allows CBF to be calculated from the temperature difference between the plates. However, the system is not able to measure CBF when temperature is greater than 39° C.
Selective Brain Cooling
How to cool is beyond the scope of this chapter, but a brief mention is made of selective brain cooling because systemic hypothermia, the method used in major clinical trials, is limited by the time to target temperature, the depth of hypothermia, and complications. Selective brain cooling may solve these issues. A variety of devices, for example, intraventricular cooling catheters, neck pads, or cool packs applied to the dura during a craniotomy among many others, have been used to selectively cool the brain and generally they demonstrate localized cooling of the brain while maintaining relative systemic normothermia. The most promise may lie in the use of the paranasal sinuses or be derived from an understanding of how seals selectively cool their brains to reduce oxygen consumption in the brain during a dive. In addition, implantable cooling devices in the field of neuromodulation may become clinically feasible in the near future.
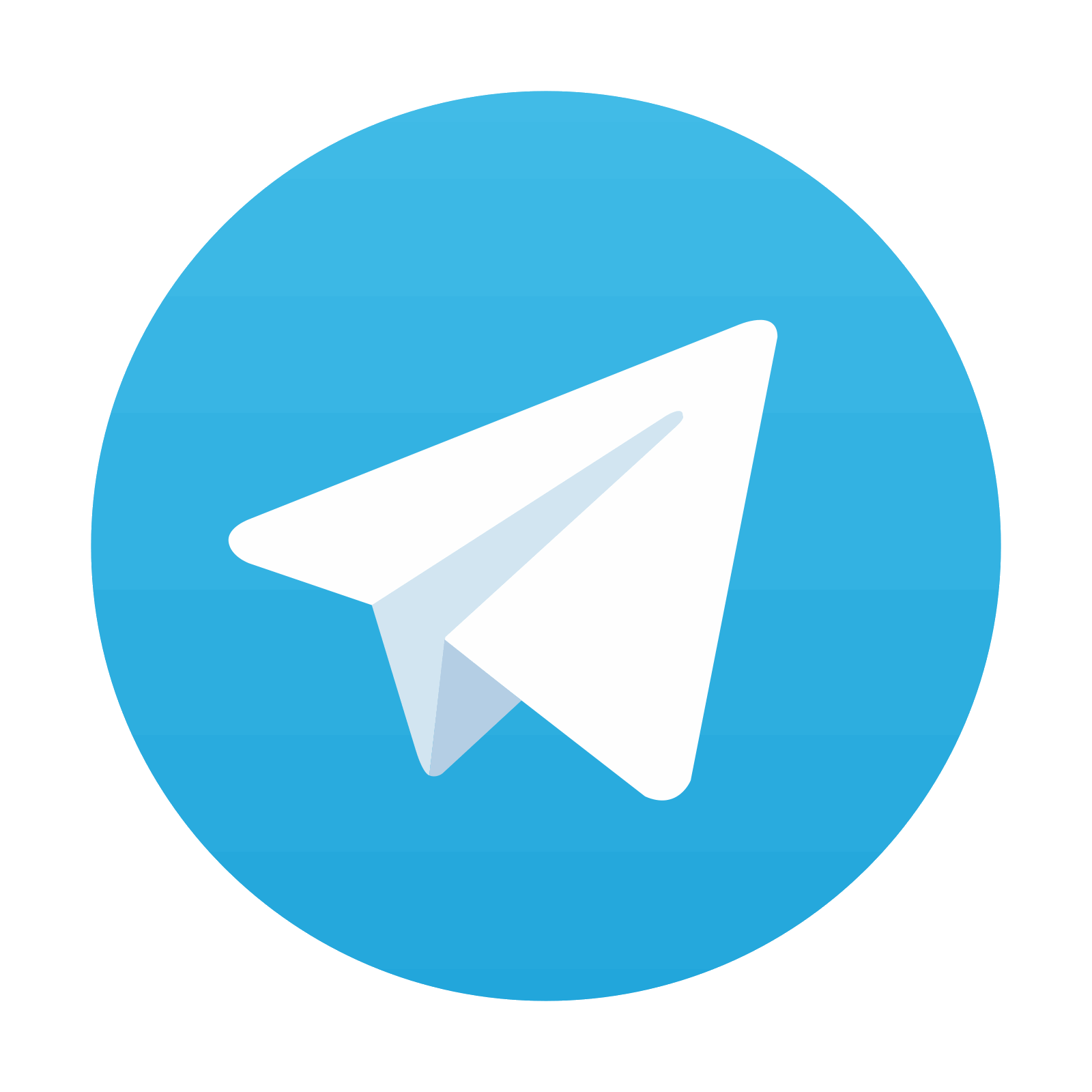
Stay updated, free articles. Join our Telegram channel
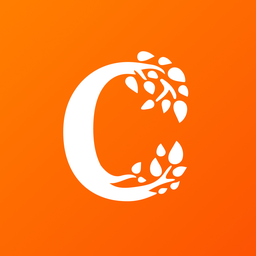
Full access? Get Clinical Tree
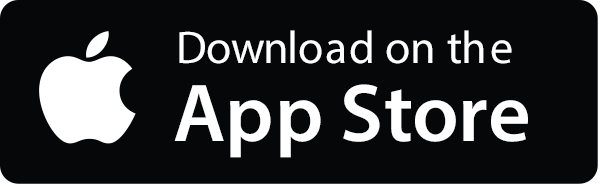
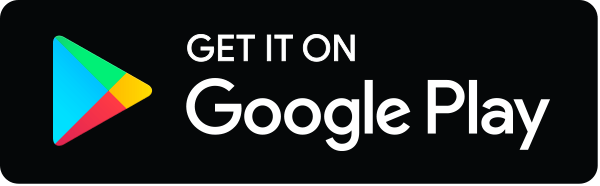