Neurons are the principal cells in the brain that process information. There is a great diversity of neuronal cell types based on morphology, chemistry, location, and connections.
The nucleus and major cytoplasmic organelles in the cell body of neurons synthesize and process proteins, which are subsequently transported to their appropriate locations within the neuron.
The axon transports molecules and conducts action potentials to presynaptic terminals to initiate communication with other neurons, which occurs at synapses.
Dendrites, multiple fine processes that extend from the neuronal cell body, together with the cell body, serve as the primary structure for the reception of synaptic contacts from other neurons.
The cytoskeleton—the inner scaffold of a neuron formed by a system of interconnected protein filaments called microtubules, intermediate filaments, and actin filaments—plays a key role in the structure of neurons and in the transport of various proteins and organelles from the cell body to axonal and dendritic processes.
Three major classes of glia—astrocytes, oligodendrocytes, and microglia—play important roles in brain function.
The blood–brain barrier, formed by tight junctions between endothelial cells of capillaries in cerebral vascular beds, allows only small lipophilic substances to enter the brain from the general circulation.
In their resting state, neurons maintain a negative electrical potential in relation to the extracellular environment. This results from differences between the intracellular and extracellular concentrations of K+, Na+, and Cl− and the relative permeability of the cell membrane to these and other ions. The energy-consuming Na+/K+ pump helps to maintain appropriate ionic gradients across the membrane.
The generation of all-or-none action potentials relies on the activities of voltage-dependent ion channels, highly specialized proteins that allow the flow of a specific ion (K+, Na+, or Ca2+) across neuronal membranes in response to changes in neuronal membrane potential.
Sodium channels are the targets of many important drugs including local anesthetics and some antiseizure medications.
The three general classes of potassium channels include voltage-gated potassium channels, calcium-activated potassium channels, and inward rectifiers.
Entry of calcium into neurons through voltage-dependent calcium channels, of which there are five major classes—L-type, N-type, T-type, P/Q-type, and R-type—is important for neurotransmitter release and activation of intracellular signaling cascades. L-type calcium channel blockers are used to treat ischemic heart disease and hypertension.
Mutations in ion channels are the cause of several neurologic disorders, including certain inherited neuromuscular disorders, epilepsy, and migraine syndromes.
It has been estimated that there are at least 100 billion neurons in the human brain, although this number in itself reveals little of the brain’s complexity. Unlike other organs, the brain contains an enormous diversity of cell types. Depending on the definition of a neuronal cell type, there may be thousands in the brain—each with their own structural, biochemical, and functional properties. Yet, the complexity of the brain as an information-processing organ extends beyond its cellular diversity. Neurons in the brain form diverse circuits that can range in scale from small local neuronal groups to long-distance projections. Many neurons function in more than one circuit and can communicate with thousands of other neurons.
Neuronal communication depends on both electrical and chemical carriers of information. The electrical mechanisms rely on the ability of each neuron to control the flow of ions across its membrane and thus to process and store information. Chemical signals are the means by which the vast majority of neurons communicate with each other. Neurons are not physically connected but are separated by a minute space, as small as 20 to 40 nm, called a synapse (2–1). The simplest synaptic arrangement involves an electrical impulse in one neuron, the presynaptic neuron, that triggers the release of a chemical substance, or neurotransmitter, which diffuses across the synaptic cleft and binds to specific receptors on another, the postsynaptic neuron. The binding of a neurotransmitter to its appropriate receptors precipitates changes in the electrical activity of the postsynaptic neuron, which in turn leads to the release of a neurotransmitter and further interneuronal communication. In a small fraction of cases, neurons physically connect with one another so that direct electrical transmission between the neurons can occur.
2–1
Electron micrograph of excitatory synapses. Each excitatory synapse forms an asymmetric junction, which exhibits a prominent postsynaptic thickening called the postsynaptic density (red). Axon terminals opposite the postsynaptic density contain small, spherical vesicles. One terminal (green) can be seen making contact with a dendritic spine (yellow). Most excitatory synapses are surrounded by astroglial processes (blue). (Reproduced with permission from Witcher MR, Kirov SA, Harris KM. Plasticity of perisynaptic astroglia during synaptogenesis in the mature rat hippocampus. Glia. 2007;55(1):13–23.)

Neurons in the brain can form thousands of synapses with other neurons; extreme examples are a Purkinje cell in the cerebellum or a monoamine-containing cell in the brainstem that may form more than 100,000 synapses. Overall, in a single human brain, there are likely to be more than 100 trillion synapses. Complex processes of brain development that are not fully understood result in connections among neurons—some local, some over long distances—that are both highly specific and highly plastic.
The overall patterns of neural connectivity in the mammalian central nervous system (CNS) are dictated by a complicated set of genetically programmed interactions. Nevertheless, both spontaneous neural activity and neural activity that occurs in response to stimulation during gestation and throughout life have profound influences on the fine-tuning of an individual’s pattern of synaptic connections. Although there are finite critical periods of early postnatal development during which the pattern of neural connectivity in some circuits is markedly influenced by experience, the adult brain is far more plastic than previously thought and synaptic connectivity is modified throughout life. Thus, unlike computers, which are sometimes represented as artificial brains, the neural circuitry of the mammalian brain is not hardwired but instead constantly reacts and adapts to an ever-changing environment. The advent of optogenetics and related tools has vastly expanded the ability to study the function of precise neural circuits in the brain 2–1.
2–1 Optogenetics: Revolutionizing the Study of Brain Circuits
Major scientific advances are often driven by the development of new methodologies. One such advance over the last decade—optogenetics—has dramatically enhanced the study of the neural circuit basis of brain function. Optogenetics refers to the targeting of light-sensitive recombinant proteins to certain cells in the brain followed by optical stimulation to achieve precise spatial and temporal control over the activity of those cells.
By probing the genomes of primitive organisms, scientists discovered light-sensitive proteins, termed opsins. These opsins function normally when expressed in neurons and include light-activated ion channels and ion pumps that are targeted to membranes. One of the most important ion channel opsins is called channelrhodopsin (ChR2). When activated by blue light, it functions as a nonspecific cation channel and strongly depolarizes cells. In the absence of blue light, which normally never enters the brain, it is inert. By expressing ChR2 in neurons and exposing the neurons to very short pulses of blue light, it is possible to generate action potentials that mimic the neurons’ natural firing patterns. By targeting ChR2 (or related opsins) to a specific cell type in the brain, by use of viral gene transfer or transgenic approaches, investigators can precisely activate these cells and determine the behavioral consequences in awake behaving animals. It is also possible to activate the presynaptic terminals of ChR2-expressing neurons and thereby determine the behavioral consequences of activating different afferent inputs to a given brain region.
Of equal importance is the existence of opsins that inhibit the activity of neurons. Two examples are an inward chloride pump termed halorhodopsin (NpHR) and an outward proton pump termed Arch. NpHR and Arch are activated by yellow light and can hyperpolarize cells for prolonged periods of time. Thus, by targeting these inhibitory opsins to specific cell types, scientists can determine the behavioral consequences of inhibiting the activity of specific sets of neurons in awake behaving animals.
Optogenetics has become a standard, and ever improving, experimental tool to study the function of specified circuits in the brain. It is also being employed to gain light control over other types of proteins in the brain, for example, intracellular signaling proteins. It is even conceivable that optogenetics might eventually be applied to the human nervous system as a therapeutic modality to repair malfunctioning circuits that cause debilitating symptoms.
A related approach—termed designer receptors exclusively activated by designer drugs (DREADDs)—involves expressing in the brain a modified G protein–coupled receptor that signals through a particular G protein (eg, Gq, Gi) (Chapter 4). The receptor is modified to respond to a synthetic ligand (eg, clozapine N-oxide) that has minimal effects on endogenous receptors. In this manner, it is possible to experimentally activate a particular signaling pathway in affected neurons. While this approach lacks the temporal resolution of optogenetics, it offers other advantages.
Although the neuron is the critical cell type for communication in neural networks, essential roles are played by glial cells. The brain contains three major classes of glia: astrocytes, oligodendrocytes, and microglia. Astrocytes have the most diverse functions, which include maintenance of the extracellular milieu (the composition, including ion concentrations, of extracellular fluid in the brain) for healthy neuronal function, metabolism of certain neurotransmitters, and formation of the blood–brain barrier. Astrocytes also are critical in the CNS response to injury. Oligodendrocytes produce myelin sheaths that encase axons and facilitate the conduction of action potentials. Microglia, together with lymphocytes and macrophages that migrate to the CNS from the periphery, are the cellular components of the brain’s immune system. All types of glia elaborate soluble factors, including neurotrophic factors and cytokines, which are involved in the maintenance of the nervous system and in its adaptation to changes in the environment (Chapter 8). Indeed, astrocytes are important in modulating the process of synaptic transmission. Glia also are key components in guiding the migration of growing neurons during development.
This chapter focuses on the basic features of neurons and glia and on the electrical excitability of neurons. The molecular and cellular basis of synaptic transmission and signal transduction are covered in subsequent chapters.
Neurons are highly asymmetric (polarized) cells that have three major components: a cell body (also known as a soma or perikaryon), a single long process called an axon, and a varying number of branching processes known as dendrites 2–2. Aggregations of neuronal cell bodies form the gray matter of the brain (named for its appearance on freshly cut sections). Axons make up the white matter, whose appearance results from the myelin sheaths that insulate many axons. Although neurons share a common set of features, they have a variety of sizes and shapes 2–3 and serve very different functions within the networks in which they operate.
2–2
Principal features of a typical vertebrate neuron. Dendrites, which are the primary sites of synaptic contact, receive most of the incoming synaptic communication. The cell body contains the nucleus and is the site of gene transcription. The axon transmits information and often has multiple branches, the terminals of which form synapses with the dendrites of other neurons. (Reproduced with permission from Kandel ER, Schwartz JH, Jessell TM. Principles of Neural Science, 4th ed. New York: McGraw-Hill; 2000.)

The cell body contains the nucleus and major cytoplasmic organelles such as the rough and smooth endoplasmic reticulum (ER) and Golgi apparatus 2–4. It is primarily responsible for synthesizing and processing proteins, which are subsequently transported to their appropriate locations within the neuron. The nucleus contains genomic DNA that is transcribed into mRNA; mRNA is exported from the nucleus to the cytoplasm where it is translated into protein on ribosomes (Chapter 4). Although most mRNA remains in the cell body, some of it is transported to dendrites and axon terminals. Polyribosomes, which are multiple ribosomes arrayed on mRNA, and ER are found in dendrites, often right beneath synapses, where they presumably permit localized protein synthesis. Local control of protein synthesis may permit alterations to be made to specific synapses within a neuron, a mechanism that may underlie very precise changes in neural circuit function.
2–4
Diagram and electron micrograph of intracellular organelles. The nucleus (Nu) is the site of transcription of DNA to RNA. Proteins are synthesized in the endoplasmic reticulum (ER) and subsequently transported to the Golgi apparatus (G) where they undergo further modifications before they are transported to their final destination. Also shown are mitochondria (M), the energy storehouses for the cell, and the plasma membrane (PM). (Used with permission from J Buchanan, Stanford University School of Medicine.)

The cell body is the smallest part of the neuron; the bulk of cytoplasmic volume is distributed throughout the axon and the dendritic arbor. Yet, because it must produce components that sustain the rest of the neuron, the metabolic and synthetic demands on the neuronal cell body are immense. Thus, the cell body contains large numbers of mitochondria, which are the sites of oxidative phosphorylation and provide the main form of energy (adenosine triphosphatase [ATP]) used by all eukaryotic cells.
The axon is a fine tubular process that extends from the neuronal cell body; it conducts electrical impulses from the cell body to the axon terminals (presynaptic boutons) that form the presynaptic component of a synapse. Projection neurons are those that send axons to another region of the CNS or to the periphery, as opposed to interneurons whose axons remain within the CNS region of origin. Neurons generally have a single axon, the length of which varies from less than 1 mm for interneurons to more than 1 m for motor neurons that innervate the extremities. The axons of long projection neurons typically are myelinated; those of local circuit neurons usually do not have myelin sheaths. The axon normally emerges from a region of the cell body called the axon hillock 2–2, the region of the neuron from which an action potential is most often generated. As it approaches its terminal field of innervation, an axon may branch to varying degrees, depending on the number of neurons with which it makes synaptic contact. Axons also may give rise to recurrent collaterals that innervate other neurons in their vicinity and thereby serve feedback regulatory functions.
Dendrites are multiple fine processes that extend from the neuronal cell body and, together with the cell body, serve as the primary structure for the reception of synaptic contacts from other neurons. The geometry of dendritic arbors can be very complex and indeed beautiful (see 2–3). The precise location and extent of a dendritic arbor determine the role of a cell in a network. Many types of neurons have discrete spines protruding from their dendrites 2–5. Such spines typically receive the major excitatory inputs directed to their respective cells. Moreover, the spines are thought to structurally and biochemically isolate synapses so that each synapse serves as a small, individual unit of information processing (Chapter 3). Dendritic spines are not fixed structures; neurons regulate the number and morphology of spines, in some cases over minutes and hours, in response to neural activity and environmental signals.
2–5
Image of dendritic branches of a living hippocampal pyramidal neuron reveals a high density of dendritic spines. This cell expresses green fluorescent protein (GFP) and was imaged using laser confocal fluorescence microscopy. (Used with permission from Virginie Biou, Stanford University School of Medicine.)

The main functions of the dendritic tree include the reception, processing, and integration of incoming synaptic communications. Dendrites are both electrically and biochemically quite complex. Dendrites, like axons, contain voltage-dependent ion channels and thus can fire action potentials and actively propagate information to the soma. They also contain a wide variety of intracellular signaling molecules (Chapter 4) that are activated during synaptic communication and alter neuronal function.
The cytoskeleton, which represents the inner structure, or scaffold, of a neuron, is formed by a system of interconnected molecular filaments termed microtubules, intermediate filaments, and actin filaments. Microtubules are made of polymers of tubulin, a globular protein that forms a heterodimer between α and β tubulin. Microtubules copurify with several microtubule-associated proteins (MAPs) such as tau, which have significant roles in the assembly of microtubules, in cross-linking them to other filaments, and in transport functions. Intermediate filaments of neurons, called neurofilaments, are formed by three polypeptide subunits of low, middle, and high molecular masses. Actin filaments (also called microfilaments) are made of actin, a globular protein that self-assembles into a linear polymer. Microtubules and intermediate filaments are cross-linked to form a longitudinal scaffold for axons and dendrites. Actin microfilaments form a network underneath the entire surface membrane of the neuron; in dendrites and axons they are connected to microtubules and intermediate filaments. Actin microfilaments are heavily concentrated in dendritic spines and growth cones, both of which are dynamic structures that can respond to extracellular signals by changing shape. Many and perhaps all neurons in the CNS possess cilia. They are important for the migration of neurons during development but may also continue to serve important functions in the adult brain.
The cytoskeleton not only has important structural functions but also controls the transport of proteins between the cell body and its axonal and dendritic processes. Both fast anterograde and retrograde transport of proteins (100–400 mm per day) and slow anterograde transport (0.1–3.0 mm per day) occur in axons. Fast anterograde axonal transport involves the movement of transport vesicles, derived from the Golgi, along axonal microtubules. These vesicles contain many of the proteins necessary for the functioning of the presynaptic terminal.
The power to move vesicles along microtubules by fast axonal transport is derived from two force-generating proteins, kinesin and dynein. These proteins are ATPases that, by binding to microtubules, are stimulated to transport vesicles. Because microtubules have an intrinsic polarity, with plus ends pointing toward presynaptic terminals and minus ends pointing toward the cell body, they can serve as a compass to direct vesicle traffic. Kinesin and dynein also have an intrinsic polarity; kinesin moves vesicles only toward the plus end of microtubules and therefore is the motor protein for anterograde fast transport, and dynein moves them only toward the minus end and thus is the motor protein for retrograde fast transport. Retrograde axonal transport functions to return various membrane molecules to the soma for elimination and is also important for communicating information from nerve terminals to the soma.
The molecular mechanisms responsible for slow axonal transport and for the transport of proteins to dendrites are not well understood. Slow axonal transport appears to utilize the proteins that comprise the cytoskeleton itself—a dynamic rather than a static structure that is continually renewed. Proteins that are specifically directed toward dendrites rather than axons must be guided by molecular recognition signals that dictate the direction of transport. The dendritic cytoskeleton is different from that of axons. Unlike axons, in which the polarity of microtubules is fixed, dendrites have microtubules whose polarity varies because equal numbers of microtubules are oriented in each direction. The proteins associated with dendritic microtubules also differ from those of axonal microtubules; MAP2 is found only in dendrites and in the cell body, whereas the protein tau is found almost exclusively in axons. Perhaps these differences help guide the transport of specific proteins to specified sites within neurons.
A synapse is a specialized structure involved in the transmission of information from one neuron to another. Synapses typically are composed of a single presynaptic element—the presynaptic terminal or bouton of an axon—and a postsynaptic element, which for excitatory synapses is often a dendritic spine. Although the synaptic cleft lies between these two elements, it is incorrect to think of the cleft as empty space that separates two independent structures. Instead, the presynaptic and postsynaptic elements of a synapse are tightly bound to one another and to the extracellular matrix (composed of proteoglycans, other polysaccharides, and proteins) by means of numerous scaffolding proteins, such as cell adhesion molecules (CAMs), and by astrocytes (see 2–2). The synapse should therefore be considered an individual unit whose sole purpose is to transmit, process, and store information.
Most synapses in the brain utilize chemical transmission. Neurotransmitters 2–2 are typically released from presynaptic terminals, and diffuse across a synaptic cleft and bind to specialized receptor proteins on postsynaptic cells (Chapters 3 and 4). The presynaptic terminal contains specialized cellular structures that allow it to remain, to a certain extent, metabolically and functionally independent from the neuronal cell body. It contains large numbers of mitochondria to provide energy, enzymes to synthesize and degrade neurotransmitters, and synaptic vesicles to store substantial concentrations of neurotransmitters while waiting for a signal to be released. The postsynaptic dendritic membrane is markedly enriched with appropriate neurotransmitter receptors and elaborate intracellular signaling machinery.
2–2 Identification of Neurotransmitters in the Brain
Our understanding of the molecular basis of neuropharmacology is significantly dependent on our ability to identify neurotransmitters in the mammalian brain. In theory, a substance that is released in response to stimulation of a neuron and that is capable of generating a measurable postsynaptic response, electrophysiologic or biochemical, might be classified as a neurotransmitter. However, to help elucidate the extraordinary complexity of neural signaling, more explicit criteria have been used to identify neurotransmitters.
Localization
A putative neurotransmitter must be localized to presynaptic terminals (or in some cases to dendrites or somas) in specific neural pathways 2–3. Techniques for such localization include immunohistochemical staining and biochemical analysis of regional concentrations of the substance under study. The localization of enzymes required for the synthesis, degradation, or uptake of the substance helps to confirm the identification of a neurotransmitter.
Release
Classically, neurotransmitters are released in a Ca2+-dependent manner, which can be established, for example, by depleting Ca2+ or blocking Ca2+ entry into neurons (Chapter 3). In an intact brain, it can be determined whether stimulation of a pathway causes the release of a candidate neurotransmitter as measured in extracellular fluid by techniques such as microdialysis or, in the case of some substances, by electrochemical detection.
Synaptic mimicry
The action of a suspected neurotransmitter should be mimicked by exogenous application of the substance. Such mimicry can be accomplished in vitro by application of the substance to reduced brain preparations, or in vivo by means of microiontophoresis. The actions of the substance can be evaluated by means of electrophysiologic, biochemical, or behavioral measurements.
Synaptic pharmacology
Neurotransmitters act on receptors for which there may exist pharmacologic antagonists. Thus, if the action of a synaptically released substance is blocked by a selective receptor antagonist, the identity of the neurotransmitter is strongly suggested. Receptor agonists also may be used to demonstrate synaptic mimicry but may provide inaccurate results because several receptor subtypes can be coupled to the same postsynaptic effector mechanisms.
2–3 The Many Faces of Synaptic Transmission
A synapse was initially thought to involve a postsynaptic dendrite or cell body innervated by a presynaptic nerve terminal A. This classic definition described a synapse both structurally and functionally in terms of presynaptic and postsynaptic elements. Although such synapses, which can be termed axodendritic or axosomatic, are widespread in the CNS and may represent the predominant mode of synaptic transmission involving excitatory and inhibitory amino acids, we have learned that many additional types of synaptic transmission occur in the brain.
Axoaxonic synapses occur when neurotransmitters released from one nerve terminal act on receptors located on other nearby nerve terminals B. Such nerve terminals may be functionally presynaptic in one synapse but functionally postsynaptic in another. Neurotransmitters also can act by means of autoreceptors located on the same terminals that release them C. Neurotransmitters can be released from cell bodies or dendrites (eg, nitric oxide, endogenous cannabinoids) and diffuse to act on neighboring nerve terminals D, resulting in a dendroaxonic synapse.
It is likely that several types of synaptic relationship coexist in most regions of the brain. Consider a hypothetical situation in which an excitatory amino acid nerve terminal innervates a dendritic spine by means of a classic axodendritic synapse E. In addition to acting on the dendritic spine, the released glutamate can affect further glutamate release by acting on autoreceptors at its own nerve terminals. Glutamate release is further modulated by nearby γ-aminobutyric acid (GABA)-ergic nerve terminals, which function as axoaxonic synapses with the glutamatergic terminals. Released monoamines or endogenous cannabinoids modify glutamate release from glutamatergic nerve terminals by means of actions on presynaptic receptors and modify postsynaptic responses to glutamate through actions on receptors near or on the dendritic spines.
Synapses that involve the innervation of a postsynaptic dendrite by a presynaptic nerve terminal represent just one anatomic arrangement of chemical synapses. Other arrangements, in particular, where substances are released by dendrites that act on nerve terminals, are described in 2–3. Overall, chemical synapses are predominant in the CNS but do not represent the only form of synaptic transmission. In a small minority of cases, neurons are connected by means of a gap junction rather than separated by an intervening space. Gap junctions, which are formed by a large number of tightly packed proteins (connexons), produce so-called electrical synapses that permit electrical currents to flow directly between cells.
Every heartbeat, every nerve impulse, every movement, and every thought is critically dependent on the tightly controlled and precisely timed flow of ions across cell membranes. A disruption of this flow, for example, by the puffer fish poison tetrodotoxin, can be fatal. Ion channel abnormalities are responsible for many human diseases (now called channelopathies). Mutations of ion channels can result in severe deficits in neuromuscular functioning, including episodic ataxias and paralyses, myotonia, and long QT syndrome of the heart as well as seizure disorders 2–1. Moreover, ion channels are also the targets of widely used and efficacious pharmacologic agents. For example, phenytoin and carbamazepine, which are used to treat epilepsy, act by altering Na+ channel kinetics. Lidocaine and procaine, common local anesthetics, block voltage-gated Na+ channels and prevent the conduction of nerve impulses that signal the occurrence of tissue damage and therefore pain. An awareness of ion channel structure and function is crucial for understanding neuropharmacology and neuronal disease processes.
Family | Disease and Symptoms |
---|---|
|
|
|
|
|
|
|
|
|
|
|
|
|
|
|
|
An animal’s nervous system receives information from the environment, integrates this information with past experience, and creates a behavioral response that promotes the survival of the organism. Sensory neurons receive information from both the external environment—for example, sounds and sights—and the internal environment, such as the sensation of hunger or the stretch of a muscle, and relay messages to other neurons. Neurons that receive these messages are responsible for directing appropriate signals to various locations that may include muscles, internal organs, glands, or other neurons. The swift communication of these signals typically benefits an organism. It can, for example, enable a prey’s rapid response to the appearance of a predator, or produce a reflexive postural correction that is necessary to prevent a fall.
Neurons convey signals rapidly by alternately maintaining and varying their electrical potentials in relation to the extracellular environment. All neurons maintain a negative electrical potential (also known as a “membrane potential”) relative to their extracellular environment. This negative potential provides a driving force for charged particles; in the absence of other forces, positive charges tend to be drawn into the cell, and negative charges tend to be repelled from the cell. When a neuron is activated by an external stimulus such as a chemical signal from another neuron, or by an event in the environment, it may depolarize; that is, its electrical potential may become less negative relative to the extracellular milieu. A neuron may, for example, depolarize from −70 to −50 mV. If a neuron undergoes significant depolarization, it may generate an action potential—a brief, all-or-none depolarization and repolarization of the membrane potential 2–6. Such substantial, rapid depolarization will often stimulate a neuron to release neurotransmitters and thus convey information to other cells through chemical signals; for example, a signal conveyed to muscle cells may cause the contraction of a muscle, and a signal conveyed to an internal organ may stimulate or attenuate its activity.
2–6
Membrane potential. A. As a sharp electrode penetrates a neuron, the difference in potential between the recording electrode and the bath drops from 0 to −70 mV. B. When small currents pass through the electrode, the potential of the cell changes in a passive manner. Larger currents elicit an all-or-none action potential.

Two characteristics of nerve cells contribute to their ability to maintain an electrical potential. First, different types of ions are unequally distributed across the neuronal cell membrane. Generally, the neuron’s interior contains a higher concentration of K+ and lower concentrations of Na+, Ca2+, and Cl− than does the extracellular space. These ionic gradients, which occur not only in neurons but also in most cell types,1 are maintained through ion-specific pumps that require energy. Moreover, the neuron’s interior contains many negatively charged, membrane-impermeable proteins that do not exist in the extracellular milieu.
Second, the neuronal cell membrane is differentially permeable to ions. In the resting state, most neurons are highly permeable to K+, somewhat permeable to Cl−, and very slightly permeable to Na+ and Ca2+. Because the cell membrane is a lipid bilayer, it would be completely impermeable to ions if it did not contain specialized proteins for ion transit. Ions strongly prefer interaction with the polar water molecules in intracellular and extracellular spaces to interaction with the hydrophobic lipid groups that comprise the bulk of the cell membrane. The selective permeability of the cell membrane depends on the numbers and states of its various ion channels. These neuronal properties—unequal permeability of ions across the cell membrane and unequal distribution of ions—are theoretically sufficient to maintain an electrical potential in a cell.
1Evolutionarily, the maintenance of ionic gradients may have developed from a drive to maintain an extracellular environment similar to that of seawater, where it is speculated that life began. Although higher in osmolarity than mammalian extracellular fluid, seawater possesses a similar K+ to Na+ to Cl– ratio.
Consider a very simple model of a cell. The interior of the cell (I) contains 100 mM K+A−, where A− is an impermeant anion such as a negatively charged protein. Exterior to the cell (O), the concentration of K+A− is 5 mM 2–7. To simplify this model, the effects of osmosis are ignored.
2–7
A cell model. A. If an impermeable membrane is placed between two compartments that contain different concentrations of the ionic solution K+A−, no movement of electrical charge can occur and the difference in potential between the two compartments (E) is zero. B. If the membrane becomes permeable to K+, K+ initially flows down its concentration gradient from I to O, creating an electrical potential that opposes the movement of K+. The net movement of K+ between I and O stops when the electrical force repelling K+ equals the force of the concentration gradient; at this point K+ has reached its equilibrium potential (EK), which can be estimated with the Nernst equation.

If the cell’s lipid bilayer is impermeable to both K+ and A−, no movement of ions occurs across the bilayer, and the concentration of ions on each side of the bilayer remains constant. However, if the lipid bilayer of this cell became permeable to K+, and only to K+—for example, from the opening of K+-specific ion channels in the lipid bilayer—K+ ions but not A− ions would be free to move across the lipid bilayer in both directions. Because I contains 20 times more K+ than O, many more K+ ions would be likely to travel from I to O than from O to I. Consequently, a net efflux of K+ from I to O would occur; because A− would remain impermeable, it would not cross the membrane.
As soon as K+ ions begin to move to O, however, a net positive charge develops in O because K+ has left the cell without accompanying A−. This net positive charge repels K+ from O. Thus, two tendencies act in opposition to one another: (1) the tendency of K+ to move out of I because more K+ is present in I than in O and (2) the tendency of K+ to be drawn into I because of its relative negative potential.
Eventually, these two tendencies reach an equilibrium whereby the net efflux of K+ from I (favored by the concentration gradient) is equal to the net efflux of K+ from O (promoted by the electrical potential). Only a minuscule fraction of K+ must venture from I to O to create an electrical potential strong enough to balance the tendency of K+ to leave I along its concentration gradient. As a consequence, a minuscule fraction of the A− ions in I exists without accompanying K+ ions, and this tiny separation of charge creates a negative potential of −75 mV in I relative to O. This negative charge within the cell exerts just enough force on the K+ ions that the net flow of K+ ions across the membrane is zero, despite the existing concentration gradient of K+. The transmembrane electrical potential at which this equilibrium occurs (−75 mV) can be determined by the Nernst equation2 and is termed the equilibrium potential (or the Nernst potential) for K+.
2The Nernst equation is Em = 58 log(Ko/Ki), where Em is the equilibrium membrane potential, Ko is the concentration of K+ ions on the outside, and Ki is the concentration of K+ ions on the inside. The factor of 58 in this equation derives from several chemical values (eg, gas constant, temperature) as well as the valence of each ion and the conversion of natural logarithms to base 10 logarithms.
A basic understanding of membrane potential drawn from the previous cell model can assist in understanding a model that more closely resembles a mammalian nerve cell. It involves: (1) a more complete complement of extracellular and intracellular ions and (2) more realistic ionic permeabilities. 2–2 describes several features of this cell model. First, the net total charge on each side of the cell is zero, a condition that is necessary for electrical neutrality.3 Second, the cell membrane is far more permeable to K+ than to any other ion, which is the case for most neurons at rest. All other permeabilities are described relative to the permeability of K+.
What is the electrical potential at which the net flux of charge across the cell membrane equals zero? If the cell were only permeable to K+, the membrane potential would rest at the equilibrium potential of K+, because all other ions would be trapped on one side of the cell and could not migrate to contribute to the generation of an electrical potential. However, the cell is permeable to other ions, although to a much lesser degree than it is to K+. If the membrane were permeable only to Na+, the resting potential would be at the equilibrium potential of Na+. In this case, the cell interior would develop a positive potential relative to the exterior of the cell. The positive potential in the cell interior would repel Na+ ions and balance the statistical tendency of these ions to flow down their concentration gradient and into the cell.
The true equilibrium potential for this cell model would be expected to lie somewhere between the equilibrium potentials for the various ions. The membrane permeability for each type of ion determines the relative contribution of ion on the inside and outside of the membrane to the equilibrium potential. The equilibrium potential for this cell, −68 mV, lies between the equilibrium potentials estimated for K+, Na+, and Cl−.4 Because the membrane’s permeability to K+ is so much greater than its permeability to the other ions, the neuron’s resulting membrane potential is much closer to the equilibrium potential of K+ (−75 mV) than to that of Na+ (+58 mV) or Cl− (−59 mV).
Many electrophysiologic concepts and the roles of ion channels and ion channel–targeting drugs in physiologic processes can be understood intuitively by understanding the change in membrane potential caused by changing the permeability of the membrane to different types of ions via the opening and closing of ion channels. When only one type of ion channel opens, it drives the membrane potential of the cell toward the equilibrium potential of that ion. For example, if many of the Na+ channels in this cell model were suddenly opened, causing Na+ to be three times more permeable than K+, the membrane potential would move toward the equilibrium potential for Na+ (in this case +19.6 mV). If the Na+ channels were to close suddenly, bringing the permeability ratio of Na+ to K+ back to its original value of 0.01, the membrane potential would return to −68 mV in response to the efflux of K+ through the many open K+ channels.
It also is important to emphasize that the equilibrium potential for a particular ion depends on the relative concentrations of that ion inside and outside the cell, which can vary considerably among different cell types and tissues. For example, the equilibrium potential for Cl− can range from −60 to −90 mV.
3Note that electrical neutrality cannot be achieved because a separation of charge persists across the cell membrane. It is also important to note that ionic concentrations in 2–2 are given in millimolar values, although a resting potential in a normal cell of –75 mV can be produced by a femtomolar net separation of charge. Moreover, both the cytoplasm of the cell and the extracellular milieu are electrically neutral, having an equal number of positive and negative charges; the difference in charge exists across the cell membrane, which acts as a capacitor.
4This can be calculated using the Goldman–Hodgkin–Katz equation. For details, see Hille (2001).
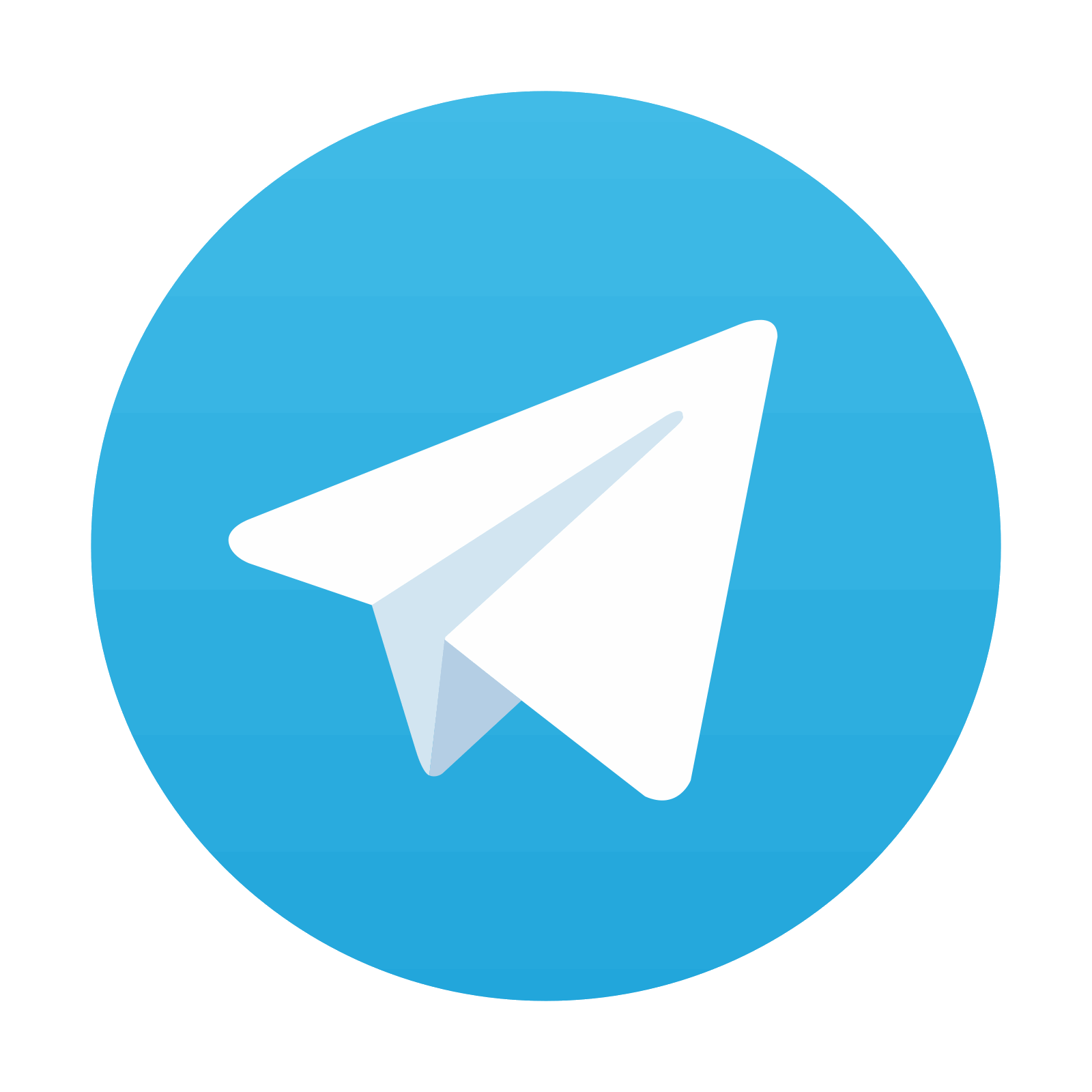
Stay updated, free articles. Join our Telegram channel
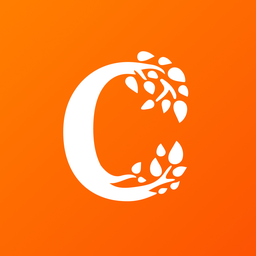
Full access? Get Clinical Tree
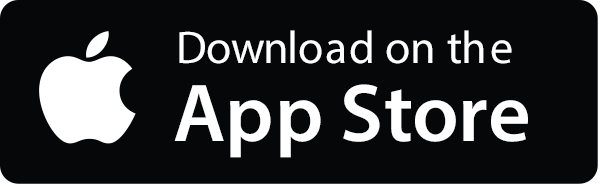
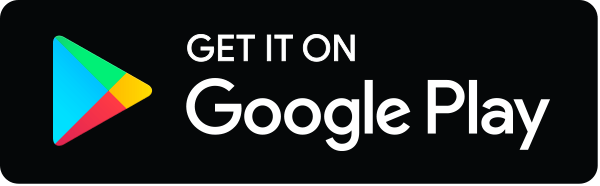
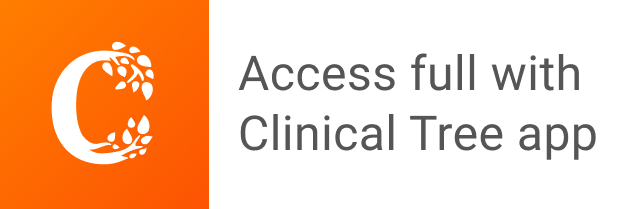