Introduction
Cerebral microdialysis (MD) is a well-established laboratory tool that is being increasingly used as a bedside monitor to provide online analysis of brain tissue biochemistry during neurointensive care. MD samples substances present in brain extracellular fluid (ECF) and has opened new avenues to monitor the injured brain. Because MD measures changes at the cellular level, it is an attractive technique to detect and monitor cerebral hypoxia, ischemia, and other causes of cellular dysfunction. To date MD has been predominantly applied to patients with traumatic brain injury (TBI) and subarachnoid hemorrhage (SAH) and has increased understanding of the pathophysiology of these conditions. Studies also have addressed its potential role as a clinical monitoring technique that can guide individualized and targeted therapy after brain injury. Future studies must identify the effect of therapeutic maneuvers on brain tissue biochemistry and establish the relationship between MD variables and outcome.
History
The first use of the MD technique was in 1966 when Bito inserted membrane-lined sacks containing 6% dextran into the cerebral hemispheres of dogs and analyzed the fluid that they contained for amino acid levels when they were removed 10 weeks later. During the 1970s Delgado developed the “dialytrode” device, which was refined later and simplified by Tossman and Ungerstedt into the “microdialysis” method. This initially was used to study neurochemical processes in animals, but in 1990, the first application in the human brain was described. The use of cerebral MD was popularized following the introduction of commercially available microdialysis catheters and a bedside analyzer (CMA 600, CMA Microdialysis, Solna, Sweden) and was first used as a neurochemical monitoring tool in neurocritical care in 1992. Devices for microdialysis in the human brain were CE-marked according to the European Medical Device Directive in 1995 and approved by the U.S. Food and Drug Administration in 2002.
Today MD is a well-established research tool but it has not yet become widely established as a routine clinical monitor. However, some centers that have extensive experience of its use as a research tool are beginning to incorporate MD monitoring into clinical practice. When considering the clinical role of MD, it is important to appreciate that it contributes a single, albeit vital, component of the whole picture of the injured brain. MD should therefore be seen as a tool to explore the neurochemical features of secondary brain injury processes with other monitors as part of a multimodal monitoring technique.
Principles of Microdialysis
The principles of the MD technique have been reviewed in detail elsewhere. In brief, a MD catheter consists of a fine double-lumen probe, lined at its tip with a semipermeable dialysis membrane. The probe tip is placed into biologic tissue, in this case the brain, and perfused via an inlet tube with fluid isotonic to the tissue interstitium. The perfusate passes along the membrane before exiting through outlet tubing into a microvial designed to collect minute amounts of fluid ( Figs. 36.1 and 36.2 ). The microvials are removed regularly, usually every hour, and placed in a bedside analyzer, which measures and records the changing brain tissue chemistry. Subsequently the samples can be analyzed off-line for other substances.


MD is based on the principle of diffusion of water-soluble substances across the semipermeable MD membrane driven by their concentration gradient. Molecules at high concentration in the brain ECF pass into the perfusate with minimum passage of water, and because the perfusate flows along the membrane and is removed at a constant rate, the concentration gradient across the membrane is maintained. In this way the MD catheter acts as an artificial blood capillary with the perfusate gradually equilibrating to the composition of ECF. The concentration of substrate in the collected fluid (the microdialysate) depends in part on the balance between substrate delivery to, and uptake or excretion from, the ECF ( Fig. 36.3 ). This simple concept provides a powerful technique in which any molecule small enough to pass across the membrane can be sampled.

Unless there is total equilibration across the dialysis membrane, the concentration of a given molecule in the microdialysate will be lower than its concentration in the brain ECF. The proportion of the true ECF concentration collected in the microdialysate is termed the relative recovery and is dependent on many factors, including dialysis membrane pore size, membrane area, perfusate flow rate, and diffusion properties of the substance. Because of the many variables that affect the relative recovery, it is essential to consider the sampling methods and materials used when comparing measured MD values. In clinical practice the most commonly used system comprises a catheter that is 10 mm in length with a 20-kDa (CMA 70, CMA Microdialysis, Solna, Sweden) or 100-kDa (CMA 71, CMA Microdialysis, Solna, Sweden) molecular weight cutoff, perfused with commercially available physiologic perfusion fluid (Perfusion Fluid CNS, CMA Microdialysis, Solna, Sweden) at a rate of 0.3 µL/min. Commonly measured bedside MD variables are reliably captured using a 20-kDa molecular weight cutoff catheter, whereas 100-kDa cutoff catheters allow investigation of higher molecular weight biomarkers. For the more clinically relevant MD variables, 100-kDa catheters have equivalent recovery to 20-kDa cutoff catheters, and many centers now use the higher cutoff catheters as a routine. A perfusate flow rate of 0.3 µL/min allows hourly sampling at the bedside, striking a balance between adequate microdialysate volume and acceptable recovery rates. Higher flow rates permit more frequent sampling but at the cost of lower concentration of measured substances in the microdialysate. A standard flow rate of 0.3 µL/min and a 10-cm catheter achieves a recovery rate in excess of 70% for routinely measured metabolites. By using lower perfusate flow rates it is possible to approach 100% recovery and measurement of the absolute concentration of a substance in brain ECF.
Although hourly MD sampling rates are adequate for most clinical purposes, continuous sampling would be advantageous when rapid and transient changes in brain metabolite levels need to be determined. A continuous MD method has been described for research purposes but it is not yet sufficiently developed for clinical application. This system incorporates continuous flow of dialysate into a glucose and lactate analyzer that uses flow-injection dual-assay enzyme-based biosensors able to determine values of metabolites every 30 seconds. The analyzer can detect changes 9 minutes after an event has occurred, and the temporal resolution is limited only by probe-to-sensor tubing length and perfusate flow rate.
Clinical Relevance of Monitoring Tissue Biochemistry in the Injured Brain
Acute brain injury frequently is exacerbated by secondary events that lead to secondary brain injury. This occurs following activation by the primary injury of an autodestructive cascade of metabolic, immunologic, biochemical, and inflammatory changes that render the brain more susceptible to systemic physiologic insults that can result in irreversible cell damage or death. Although these pathologic processes are poorly understood, they include calcium overload, increased production of free radicals, release of neurotoxic levels of excitatory amino acids (EAAs), and failing cellular metabolism. Ultimately these changes can cause cellular swelling, increase in intracranial pressure (ICP), further neuronal loss, and, if unchecked, can result in increased mortality and worsened outcome in survivors.
Secondary brain injury is a potentially modifiable cause of mortality and morbidity, and the primary aim of neurocritical care treatment of brain injury is to prevent or reduce the burden of secondary injury. Conventional intracranial monitoring techniques, such as measurement of ICP, are often “reactive” and may indicate changes only when irreversible tissue damage has already occurred. Monitoring brain tissue biochemistry through microdialysis has the potential to guide individualized therapy after brain injury, to identify impending or early onset secondary injury in some cases before there is a change in ICP, to detect cerebral compromise when ICP or cerebral perfusion pressure (CPP) is normal, and allow timely implementation of neuroprotective strategies.
Microdialysis Markers
Many substances can be measured using MD, but the key variables that are most relevant to neurocritical care can be categorized as follows :
- •
Energy-related metabolites, for example, glucose, lactate, and pyruvate
- •
Neurotransmitters, for example, glutamate, aspartate, gamma-aminobutyric acid (GABA)
- •
Markers of tissue damage and inflammation, for example, glycerol, potassium, cytokines
- •
Exogenous substances, for example, drugs
The different types of markers that can be recovered by MD highlights the utility of the technique to provide surrogate biochemical outcomes that reflect underlying pathologic mechanisms of injury. These highlighted markers represent a small fraction of those that have been recovered by MD, and a comprehensive review lists those that are less well evaluated. Several studies have addressed the recovery of protein biomarkers such as S100β, tau, beta amyloid proteins, and neurofilament. What these various biomarkers mean is still being elucidated, and the role of biomarkers in neurocritical care is discussed further in Chapter 18 .
Commercially available assays for bedside use are those for glucose, lactate, pyruvate, glycerol, and glutamate, and tentative normal values for these variables in adults are described ( Table 36.1 ). These normal values were derived from a study by Reinstrup et al., who inserted MD catheters into the frontal cortex of patients undergoing surgery for benign posterior fossa lesions and collected microdialysate samples in the postoperative course to demonstrate baseline metabolite concentrations from the uninjured human brain. Concentrations of metabolites in microdialysate samples from patients with SAH but no clinical or radiologic evidence of cerebral ischemia also have been considered to represent “normal” values. A summary of the pathophysiologic changes monitored by cerebral MD biomarkers is shown in Table 36.2 . There have been far fewer MD studies in children; these suggest there may be differences in MD findings in children compared with adults. Brain ischemia and hypoxia underlie many of the secondary cerebral insults that can cause brain damage; this results in a typical pattern of change in MD variables including an increase in glycerol and glutamate concentrations, a reduction in glucose, and an increase in the lactate/pyruvate ratio (LPR) and lactate/glucose ratio (LGR). Although an elevated LPR frequently is interpreted as a sign of cerebral hypoxia or ischemia there also are many other causes of an elevated LPR that are not related to hypoxia or ischemia. Consistent with this Nelson et al. observed that altered local biochemistry detected with MD in severe TBI patients represented long-term metabolic patterns and was weakly correlated with ICP and CPP; that is, metabolic changes other than those related to pressure or flow can influence MD findings.
Microdialysate Concentration | Normal Value ± SD Reinstrup et al. | Normal Value ± SD Schulz et al. |
---|---|---|
Glucose (mmol/L) | 1.7 ± 0.9 | 2.1 ± 0.2 |
Lactate (mmol/L) | 2.9 ± 0.9 | 3.1 ± 0.3 |
Pyruvate (µmol/L) | 166 ± 47 | 151 ± 12 |
Lactate/pyruvate ratio | 23 ± 4 | 19 ± 2 |
Glycerol (µmol/L) | 82 ± 44 | 82 ± 12 |
Glutamate (µmol/L) | 16 ± 16 | 14 ± 3.3 |
Microdialysis Variable | Biomarker for: | Comments |
---|---|---|
Low glucose |
|
|
Increased LPR |
|
|
Increased glycerol |
|
|
Increased glutamate |
|
|
Markers of Glucose Metabolism
Tissue hypoxia often is the final common pathway for cellular damage after acute brain injury, and the most commonly investigated substances assayed by MD relate to the aerobic and anaerobic metabolism of glucose. The determinants of cerebral extracellular glucose concentration are complex and depend on peripheral blood glucose concentration, local capillary blood flow, and brain cell uptake of glucose. A particular advantage of cerebral MD monitoring after brain injury is its ability to assess not only the cerebral delivery of glucose but also its use.
The energy demands of the brain are met by the metabolism of glucose, and a continued supply is vital to maintain cell integrity. Microdialysate glucose levels are reduced in patients following TBI, and a concentration consistently less than 0.66 mmol/L in the first 50 hours post injury is associated with poor outcome. The etiology of this low glucose concentration is likely to be multifactorial. In the acute period after TBI there is typically a reduction in oxidative metabolism and increase in glucose metabolism. Extremely low brain ECF glucose is observed during periods of severe hypoxia or ischemia after TBI and SAH, and associated with a brain tissue oxygen (P bt O 2 ) of less than 1.3 kPa (10 mm Hg). However, there is a poor correlation between positron emission tomography (PET)–defined ischemia and low MD glucose concentration suggesting that in some cases at least, low MD glucose concentration may be associated with hyperglycolysis rather than decreased supply of glucose and oxygen because of reduced cerebral perfusion.
Glucose is taken up into neurons and glia and initially metabolized to pyruvate by glycolysis. When there is adequate oxygen delivery and tissue oxygenation, pyruvate enters the citric acid cycle and ultimately is metabolized to carbon dioxide and water with the generation of adenosine triphosphate (ATP). During ischemia, pyruvate is diverted into the anaerobic pathway and is metabolized to lactate. The measurement of ECF lactate and pyruvate concentrations therefore provides information about the extent of anaerobic glycolysis. However, the absolute value of lactate within the brain ECF is not in itself indicative of the degree of anaerobic metabolism for several reasons. The production of lactate depends on a continued supply of glucose, which may fail during complete ischemia, and the increased levels of glutamate and potassium that are associated with ischemia drive astrocyte lactate production and hyperglycolysis. An increase in ECF glutamine may reflect this accelerated astrocyte metabolism and be found in vulnerable rather than already ischemic tissue. In addition, lactate can act as a metabolic substrate to sustain increased energy needs through the astrocyte-neuron lactate shuttle. To correct for the variable sources of ECF lactate and the dynamic variation in glucose delivery, the LPR and LGR have been used as more accurate markers of anaerobic metabolism. Because lactate and pyruvate have very similar molecular weights, the LPR is independent of catheter recovery in vivo. The LPR is therefore a robust and reliable marker of cell energy dysfunction and is the most widely monitored MD variable after brain injury.
In the human brain, worsening hypoxia, ischemia, or edema can lead to an increase in the LPR, which is associated with severe reductions in P bt O 2 and increases in PET-measured oxygen extraction fraction. An increase in LPR above established thresholds (20-25) is associated with poor outcome after TBI and SAH, and traditionally is assumed to indicate tissue hypoxia or ischemia. However, it has proved difficult to establish the tissue hypoxic threshold for a raised LPR, and it is increasingly apparent that anaerobic glycolysis may occur from ineffective utilization of delivered oxygen because of mitochondrial failure, among other causes. For example, Vespa et al. compared MD variables with PET-derived measurement of glucose and oxygen metabolism in 19 patients with severe TBI. There was a 25% incidence of metabolic crisis, defined in this study as LPR greater than 40, but only a 2.4% incidence of PET-defined ischemia. It was concluded that these data indicate that the LPR is a reliable marker of cellular dysfunction and of inadequate substrate delivery. An elevated LPR therefore may be classified as type I (ischemic), in which the pyruvate is decreased and there is a marked increase in lactate, or type II (nonischemic hyperglycolysis), in which the pyruvate is normal or increased. In a type I LPR elevation there is oxygen and glucose lack, whereas in type II LPR elevation there is mitochondrial failure or failure of effective use of delivered oxygen and glucose. In animal models of TBI, average LPR has a strong correlation with total injury volume on neuropathologic examination, confirming the value of LPR monitoring in the neurocritical care unit (NCCU).
Markers of Tissue Damage
Failure of cellular metabolism results in disruption of cell membrane function that then leads to intracellular influx of calcium, induction of phospholipase, and ultimately to degradation of cell membranes. This results in release of phospholipids and, after enzymatic breakdown, free fatty acids and glycerol into the brain ECF. Glycerol is reliably recovered from the ECF and is therefore a useful MD marker of tissue hypoxia and cell damage. The degree of the MD glycerol elevation associated with pathology (e.g., hypoxia or ischemia) may be dramatic, with four- or eightfold increases recorded in severe or complete ischemia respectively. High levels of interstitial glycerol are associated with an unfavorable outcome after TBI and indicate the severity of parenchymal damage. Cerebral MD glycerol concentration typically is elevated in the first 24 hours after severe TBI, presumably as a result of the primary injury, and then exponentially declines during the ensuring 3 days. Clausen et al. observed that P bt O 2 less than 1.3 kPa (10 mm Hg) and CPP less than 70 mm Hg were associated with elevated mean cerebral MD glycerol levels, although individual episodes below the same thresholds also occurred without an increase in MD glycerol concentration. In this study mean MD glycerol concentration was similar in patients with favorable and unfavorable outcomes. The interpretation of elevated glycerol levels after TBI therefore requires further validation. Glycerol also is able to leak through a damaged blood brain barrier (BBB) and cause spuriously high brain ECF glycerol concentration because of the high levels of plasma glycerol that occur due to stress-induced triglyceride degradation or the administration of exogenous glycerol. To help distinguish a compromised BBB effect from a true intracerebral event, systemic glycerol concentration can be simultaneously measured via an MD catheter placed in the abdominal subcutaneous adipose tissue.
Excitotoxicity
Ischemia, TBI, SAH, and other pathologies can lead to cell depolarization and release of EAAs such as glutamate and aspartate. Excitotoxicity is one of several mechanisms implicated in neuronal injury, and there was early enthusiasm about using MD to measure glutamate because animal studies demonstrated raised glutamate concentration in global cerebral ischaemia and TBI. Subsequent studies in humans also demonstrated an association between increased MD glutamate concentration and poor outcome after TBI and SAH. For example, Staub et al. observed 30-fold increases in ECF glutamate, aspartate, and GABA in SAH patients with unfavorable outcome at 3 months compared with those with favorable outcome. The increased concentration of EAA in patients with poor outcome followed a biphasic course, with maximal concentrations on the first to second, and seventh, days after the insult. It is likely that the early increase represents the degree of initial injury and the later increase the development of vasospasm-related ischemic injury. Prolonged elevation of MD glutamate also is observed after severe TBI and is associated with poor outcome. High MD-glutamate levels have been correlated with clinical events involving hypoxia, ischemia, reduced P bt O 2 , and low CPP. The role of glutamate in excitotoxicity after TBI has been challenged, and there are multiple causes of increased ECF glutamate concentration after brain injury. Nevertheless, recent studies suggest glutamate levels may provide useful information in some patients. For example, Timofeev et al. found that MD glutamate was associated weakly with mortality in 223 severe TBI patients but that this association disappeared after multivariable analysis. Chamoun et al. observed two patterns of glutamate elevation after TBI: (1) glutamate levels tended to normalize over the monitoring period (120 hours); and (2) glutamate levels tended to increase with time or remain abnormally elevated. Pattern 1 patients had a lower mortality rate (17.1% versus 39.6%) and a better 6-month functional outcome among survivors (41.2% versus 20.7%) compared to pattern 2. In addition glutamate levels greater than 20 mmol/L were associated with a nearly twofold increase in mortality.
Variability of Measured Metabolites
After brain injury there are wide variations in MD variables over time between different subjects and within individuals. These are likely to represent the changing levels of metabolic activity within the injured brain but make it difficult to interpret single MD measurements or measurement obtained in isolation. Although “normal” and threshold MD values are published, cerebral MD must be seen as a trend monitor, and the information provided by MD should be interpreted with other measured variables, clinical information, or radiologic findings.
Catheter Placement
MD monitors local tissue biochemistry and reflects metabolic disturbances and neurochemical changes only in the region of the brain where the catheter is located. Differences between MD measured variables are observed in areas close to, and far away from, focal traumatic lesions. For example, Engstrom et al. found in 22 severe TBI patients that MD markers of ischemia were significantly different in tissue adjacent to a parenchymal lesion when compared with normal tissue in the contralateral hemisphere. Vespa et al. also observed regional differences in brain tissue chemistry after TBI and confirmed the importance of catheter location. In this study, there was persistent metabolic dysfunction in pericontusional tissue, both in baseline values and trends across time, compared with normal tissue. However, despite biochemical differences between “perilesional” and less-injured brain, Timofeev et al. observed that both areas showed a relationship between other physiologic variables (e.g., ICP, CPP, and P bt O 2 ) and biochemistry. In particular, reduced CPP or brain oxygen was associated with worse neurochemistry (e.g., increased LPR), although these effects were greater in perilesional tissue and when cerebrovascular reactivity was abnormal.
There are consensus recommendations on where to place MD catheters to monitor patients after TBI and SAH. The MD catheter should be placed in “at-risk” tissue (e.g., the area surrounding a mass lesion after TBI), or the vascular territory most likely to be affected by vasospasm after SAH. These locations should allow biochemical changes to be measured in the area of brain most vulnerable to secondary injury. In diffuse axonal injury catheter placement in the nondominant frontal lobe is recommended. However, others suggest that the catheter should always be placed in a normal brain region so that it can be used to indicate global cerebral metabolism. Whether white or gray matter should be monitored is another variable to consider to interpret MD results. White matter has a lower metabolic demand than gray matter and receives a smaller proportion of the cerebral blood flow. Most studies allude to white matter placement. Commercial MD catheters have a gold tip so that the catheter position can be confirmed by computed tomography scan.
The MD catheter is a parenchymal probe that usually is inserted though a cranial access device, including single-lumen bolts designed only for MD catheters or ones that contain multiple-angled lumens to transmit other parenchymal probes such as P bt O 2 or ICP sensors. The catheter also can be placed through a burr hole or under direct vision at the time of craniotomy. These techniques require the catheter to be tunneled under the skin and fixed with sutures. Although the catheter can be inserted to varying depths at any orientation, it is susceptible to migration or displacement depending on the security of the fixation.
There always is potential for tissue trauma at the time of catheter insertion. Animal and human studies demonstrate that artificially high levels of substrate that result from trauma or inflammation at the time of insertion usually abate within the first hour of placement. A “run-in” period of at least 1 hour should therefore be allowed before using MD data.
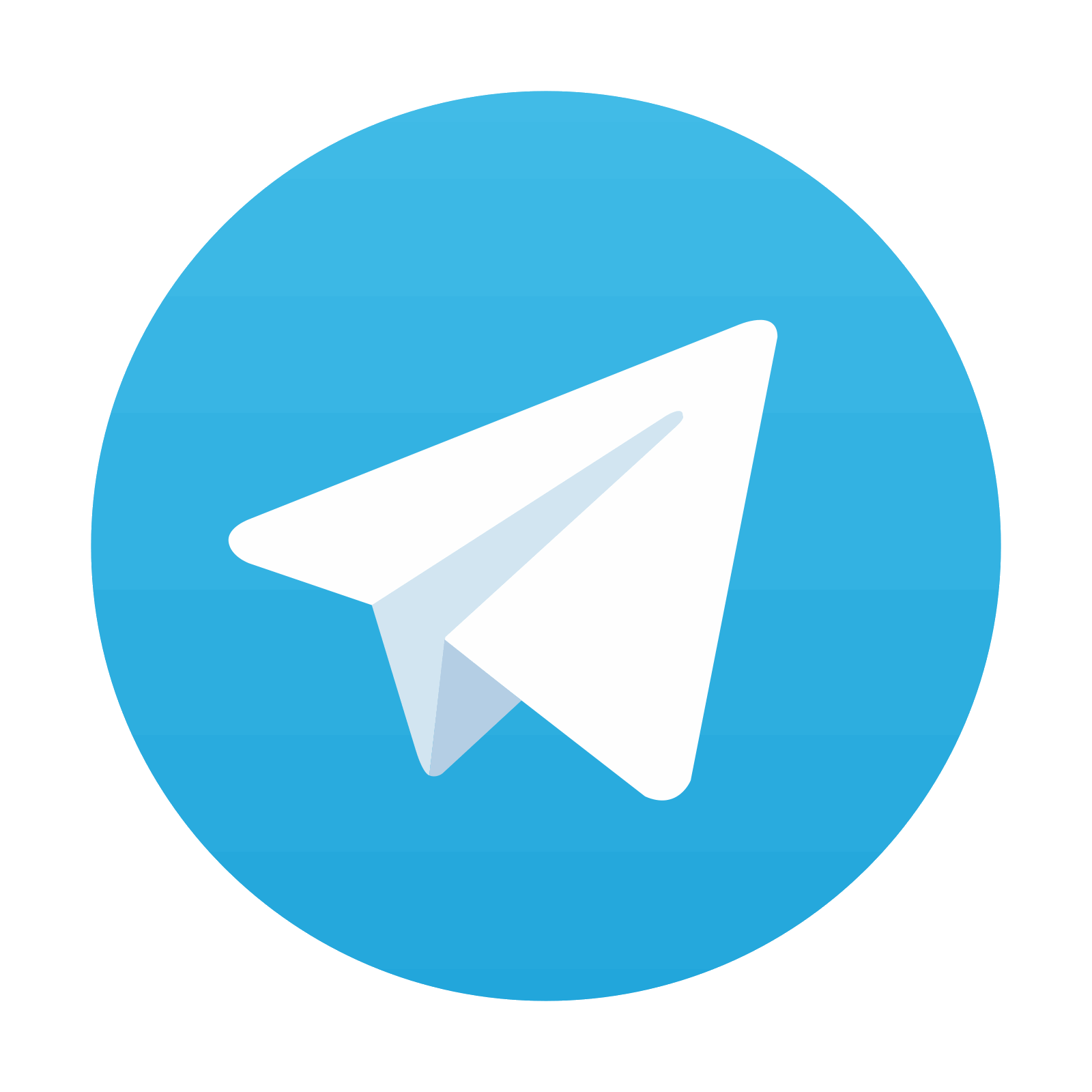
Stay updated, free articles. Join our Telegram channel
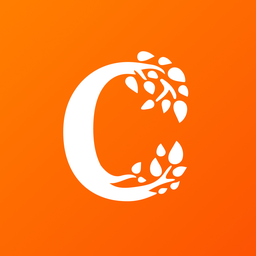
Full access? Get Clinical Tree
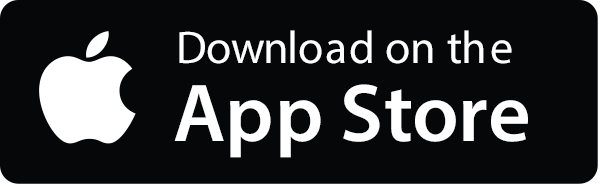
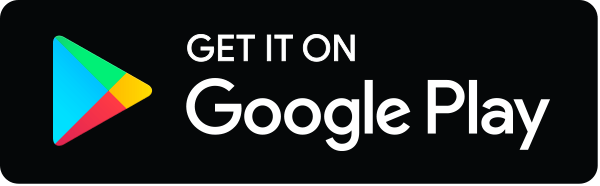