Disorders of the Autonomic Nervous System, Respiration, and Swallowing: Introduction
The human internal environment is regulated in large measure by the integrated activity of the autonomic nervous system and endocrine glands. Their visceral and homeostatic functions, essential to life and survival, are involuntary. Why the forces of evolution favored this separation from volition is an interesting question. Claude Bernard expressed this idea in sardonic terms when he wrote, “nature thought it prudent to remove these important phenomena from the caprice of an ignorant will.”
Although only few neurologic diseases exert their effects primarily or exclusively on the autonomic–neuroendocrine axis, there are numerous medical diseases that implicate this system in some way: hypertension, asthma, and certain dramatic disorders of cardiac conduction, to name some of the important ones. However, many general neurologic diseases involve the autonomic nervous system to a varying extent, giving rise to symptoms such as syncope, sphincteric dysfunction, pupillary abnormalities, erectile dysfunction, diaphoresis, cardiac dysrhythmias, and disorders of thermoregulation. Finally, in addition to their central role in visceral innervation, autonomic parts of the neuraxis and parts of the endocrine system are engaged in all emotional experience and its display, as discussed in Chap. 25.
Breathing is unusual among nervous system functions. Although continuous throughout life, it is not altogether automatic, being partly under volitional control. Current views of the central and peripheral control of breathing, and the ways in which it is altered by certain diseases are of considerable interest to neurologists, if for no other reason than respiratory failure is common in neurologic conditions such as coma, cervical spinal cord injury, and a large number of acute and chronic neuromuscular diseases. Many of these same comments pertain to the function of swallowing, which is largely automatic and continues at regular intervals even in sleep but is also initiated voluntarily. Furthermore, swallowing fails in ways similar to breathing as a consequence of neurologic diseases.
The autonomic, endocrine, and respiratory systems, although closely related, give rise to disparate clinical syndromes. This chapter deals more strictly with the autonomic nervous system and the neural mechanisms of respiration and swallowing, and the next chapter, with the hypothalamus and neuroendocrine disorders. The following discussion of anatomy and physiology serves as an introduction to both chapters.
The most remarkable feature of the autonomic nervous system is that a major part of it is located outside the brain and spinal cord, in proximity to the visceral structures that it innervates. This position alone seems to symbolize its relative independence from the cerebrospinal system. In distinction to the somatic neuromuscular system, where a single motor neuron bridges the gap between the central nervous system (CNS) and the effector organ, in the autonomic nervous system there are always two efferent neurons serving this function, one (preganglionic) arising from its nucleus in the brainstem or spinal cord and the other (postganglionic) arising from specialized nerve cells in peripheral ganglia. Figure 26-1 illustrates this fundamental anatomic feature.
Figure 26-1.
Sympathetic outflow from the spinal cord and the course and distribution of sympathetic fibers. The preganglionic fibers are in heavy lines; postganglionic fibers are in thin lines. (From Pick.)
The autonomic nervous system, from an anatomic point of view, is divided into two parts: the craniosacral, or parasympathetic, and the thoracolumbar, or sympathetic (Figs. 26-2 and 26-3). The systems differ architecturally in that the ganglion in the sympathetic nervous system is located in a contiguous and interconnected, longitudinal chain (sympathetic chain) paravertebrally, whereas the parasympathetic ganglia are distributed in proximity to the structures they innervate. Moreover, the main neurotransmitter of the postganglionic connection to the end organ is norepinephrine in the case of the sympathetic nerves and acetylcholine for parasympathetic innervation. There are exceptions with regard to the sympathetic innervation of sweat glands (sudomotor), which are cholinergic. The neurotransmitter between the pre- and postneurons throughout the autonomic nervous system, sympathetic and parasympathetic, is acetylcholine as reiterated further on. These synapses between pre- and postganglionic cholinergic nerves are not blocked by atropine (nicotinic) whereas the postganglionic impulses are blocked by atropine (muscarinic).
Figure 26-2.
The parasympathetic (craniosacral) division of the autonomic nervous system. Preganglionic fibers extend from nuclei of the brainstem and sacral segments of the spinal cord to peripheral ganglia. Short postganglionic fibers extend from the ganglia to the effector organs. The lateral-posterior hypothalamus is part of the supranuclear mechanism for the regulation of parasympathetic activities. The frontal and limbic parts of the supranuclear regulatory apparatus are not indicated in the diagram (see text). (Reproduced by permission from Noback CL, Demarest R: The Human Nervous System, 3rd ed. New York, McGraw-Hill, 1981.)
Figure 26-3.
The sympathetic (thoracolumbar) division of the autonomic nervous system. Preganglionic fibers extend from the intermediolateral nucleus of the spinal cord to the peripheral autonomic ganglia, and postganglionic fibers extend from the peripheral ganglia to the effector organs, according to the scheme in Fig. 26-1. (Reproduced by permission from Noback CL, Demarest R: The Human Nervous System, 3rd ed. New York, McGraw-Hill, 1981.)
Functionally, the two parts are complementary in maintaining a balance in the tonic activities of many visceral structures and organs. This rigid separation into sympathetic and parasympathetic parts, although useful for purposes of exposition, is physiologically not absolute. From a neurologist’s perspective, the two components are often affected together. Nonetheless, the notion of a balanced sympathetic and parasympathetic autonomic system has stood the test of time and remains a valid concept.
There are two divisions of the parasympathetic nervous system: cranial and sacral. The cranial division originates in the visceral nuclei of the midbrain, pons, and medulla. These nuclei include the Edinger-Westphal pupillary nucleus, superior and inferior salivatory nuclei, dorsal motor nucleus of the vagus, and adjacent reticular nuclei.
Axons (preganglionic fibers) of the visceral cranial nuclei course through the oculomotor, facial, glossopharyngeal, and vagus cranial nerves. The preganglionic fibers from the Edinger-Westphal nucleus traverse the oculomotor nerve and synapse in the ciliary ganglion in the orbit; axons of the ciliary ganglion cells innervate the ciliary muscle and pupillary sphincter (see Fig. 14-9).
The preganglionic fibers of the superior salivatory nucleus enter the facial nerve and, at a point near the geniculate ganglion, form the greater superficial petrosal nerve, through which they reach the sphenopalatine ganglion; postganglionic fibers from the cells of this ganglion innervate the lacrimal gland (see also Figs. 26-2 and 47-3). Other fibers originating in the salivatory nuclei are carried in the facial nerve and traverse the tympanic cavity as the chorda tympani to eventually join the submandibular ganglion. Cells of this ganglion innervate the submandibular and sublingual glands. Axons of the inferior salivatory nerve cells enter the glossopharyngeal nerve and reach the otic ganglion through the tympanic plexus and lesser superficial petrosal nerve; cells of the otic ganglion send fibers to the parotid gland.
Preganglionic fibers, derived from the dorsal motor nucleus of the vagus and adjacent visceral nuclei in the lateral reticular formation (mainly the nucleus ambiguus), enter the vagus nerve and terminate in ganglia situated in the walls of many thoracic and abdominal viscera. The ganglionic cells give rise to short postganglionic fibers that activate smooth muscle and glands of the pharynx, esophagus, and gastrointestinal tract (the vagal innervation of the colon is somewhat uncertain but considered to extend up to the descending colon) and of the heart, pancreas, liver, gallbladder, kidney, and ureter.
The sacral part of the parasympathetic system originates in the lateral horn cells of the second, third, and fourth sacral segments. Axons of these sacral neurons, constituting the preganglionic fibers, traverse the sacral spinal nerve roots of the cauda equina and synapse in ganglia that lie within the walls of the distal colon, bladder, and other pelvic organs. Thus, the sacral autonomic neurons, like the cranial ones, have long preganglionic and short postganglionic fibers, a feature that permits a circumscribed influence upon the target organ.
In organs containing smooth muscle that is innervated by parasympathetic fibers and therefore not under voluntary control, there is a parallel innervation of adjacent voluntary striated muscle by anterior horn cells. For example, the neurons that activate the external sphincter of the bladder (voluntary muscle) differ from those that supply the smooth muscle of the internal sphincter as discussed further on. In 1900, Onufrowicz (calling himself Onuf) described a discrete group of relatively small cells in the anterior horns of sacral segments 2 to 4. These neurons were originally thought to be autonomic in function, mainly because of their histologic features. There is now evidence that they are somatomotor, innervating the skeletal muscle of the external urethral and anal sphincters (Holstege and Tan). Neurons in sacral cord segments located in a region analogous to the intermediolateral cell column of the sympathetic nervous system (see later), innervate the detrusor and internal sphincter of the bladder wall. In passing, it is worth noting that in motor system disease, in which bladder and bowel functions are usually preserved until late in the disease, the neurons in the Onuf nucleus, in contrast to other somatomotor neurons in the sacral cord, tend not to be involved in the degenerative process (Mannen et al).
There are elaborate connections between supranuclear centers, mainly in the hypothalamus, to the pupillary sphincters, lacrimal and salivary glands that course the brainstem. With regard to the supranuclear innervation of parasympathetic nuclei in the sacral segments, little is known. There appear to be connections to these neurons from the hypothalamus, locus ceruleus, and pontine micturition centers but their course in the human spinal cord has not been identified with certainty.
The preganglionic neurons of the sympathetic division originate in the intermediolateral cell column of the spinal gray matter, from the eighth cervical to the second lumbar segments. Low and Dyck (1977) have estimated that each segment of the cord contains approximately 5,000 lateral horn cells and that there is an attrition of 5 to 7 percent per decade in late adult life. The axons of the nerve fibers originating in the intermediolateral column are of small caliber and are myelinated; when grouped, they form the white communicating rami as shown in Fig. 26-1. These preganglionic fibers synapse with the cell bodies of the postganglionic neurons, which are collected into two large ganglionated chains or cords, one on each side of the vertebral column (paravertebral ganglia), and several single prevertebral ganglia. These constitute the sympathetic ganglia.
Axons of the sympathetic ganglion cells are also of small caliber but are unmyelinated. Most of the postganglionic fibers pass via gray communicating rami to their adjacent spinal nerves of T5 to L2; they supply blood vessels, sweat glands, and hair follicles, and also form plexuses that supply the heart, bronchi, kidneys, intestines, pancreas, bladder, and sex organs. The postganglionic fibers of the prevertebral ganglia (located in the retroperitoneal posterior abdomen rather than paravertebrally, along the sides of the spinal column) form the hypogastric, splanchnic, and mesenteric plexuses, which innervate the glands, smooth muscle, and blood vessels of the abdominal and pelvic viscera (see Fig. 26-3).
The sympathetic innervation of the adrenal medulla is unique in that its secretory cells receive preganglionic fibers directly, via the splanchnic nerves. This is an exception to the rule that organs innervated by the autonomic nervous system receive only postganglionic fibers. This special arrangement can be explained by the fact that cells of the adrenal medulla are the morphologic homologues of the postganglionic sympathetic neurons and secrete epinephrine and norepinephrine (the postganglionic transmitters) directly into the bloodstream. In this way, the sympathetic nervous system and the adrenal medulla act in unison to produce diffuse effects, as one would expect from their role in emergency reactions.
There are 3 cervical (superior, middle, and inferior, or stellate), 11 thoracic, and 4 to 6 lumbar sympathetic ganglia. The head receives its sympathetic innervation from the eighth cervical and first two thoracic cord segments, the fibers of which pass through the inferior to the middle and superior cervical ganglia. Postganglionic fibers from cells of the superior cervical ganglion follow the internal and external carotid arteries and innervate the blood vessels and smooth muscle, as well as the sweat, lacrimal, and salivary glands of the head. Included among these postganglionic fibers, issuing mainly from T1, are the pupillodilator fibers and those innervating the Müller muscle of the upper eyelid (it connects the upper tarsus to the undersurface of the levator); there is a separate small inferior tarsus muscle that is also sympathetically innervated. The arm receives its postganglionic innervation from the inferior cervical ganglion and uppermost thoracic ganglia (the two are fused to form the stellate ganglion). The cardiac plexus and other thoracic sympathetic nerves are derived from the stellate ganglion and the abdominal visceral plexuses, from the fifth to the ninth or tenth thoracic ganglia. The lowermost thoracic ganglia have no abdominal visceral connections; their axons course rostrally and caudally in the sympathetic chain. The upper lumbar ganglia supply the descending colon, pelvic organs, and legs.
The terminals of autonomic nerves and their junctions with smooth muscle and glands have been more difficult to visualize and study than the motor end plates of striated muscle. As the postganglionic axons enter an organ, usually via the vasculature, they ramify into many smaller branches and disperse, without a Schwann cell covering, to innervate the smooth muscle fibers, the glands, and, in largest number, the small arteries, arterioles, and precapillary sphincters (see Burnstock). Some of these terminals penetrate the smooth muscle of the arterioles; others remain in the adventitia. At the ends of the postganglionic fibers and in part along their course there are swellings that lie in close proximity to the sarcolemma or gland cell membrane; often the muscle fiber is grooved to accommodate these swellings. The axonal swellings contain synaptic vesicles, some clear and others with a dense granular core. The clear vesicles contain acetylcholine and those with a dense core contain catecholamines, particularly norepinephrine (Falck). This is well illustrated in the iris, where nerves to the dilator muscle (sympathetic) contain dense-core vesicles and those to the constrictor (parasympathetic) contain clear vesicles. A single nerve fiber innervates multiple smooth muscle and gland cells.
Somewhat arbitrarily, anatomists have declared the autonomic nervous system to be purely efferent motor and secretory in function. However, most autonomic nerves are mixed, also containing afferent fibers that convey sensory impulses from the viscera and blood vessels. The cell bodies of these sensory neurons lie in the posterior root sensory ganglia; some central axons of these ganglionic cells synapse with lateral horn cells of the spinal cord and subserve visceral reflexes; others synapse in the dorsal horn and convey or modulate impulses for conscious sensation. Secondary afferents carry sensory impulses to certain brainstem nuclei, particularly the nucleus tractus solitarius, as described later, and the thalamus via the lateral spinothalamic and polysynaptic pathways.
Integration of autonomic function takes place at two levels, the brainstem and the cerebrum. In the brainstem, the main visceral afferent nucleus is the nucleus tractus solitarius (NTS). Cardiovascular, respiratory, and gastrointestinal afferents, carried in cranial nerves X and IX via the nodose and petrosal ganglia, terminate on specific subnuclei of the NTS. The caudal subnuclei are the primary receiving site for viscerosensory fibers; other less-well-defined areas receive baroreceptor and chemoreceptor information. The caudal NTS integrates these signals and projects to a number of critical areas in the hypothalamus, amygdala, and insular cortex, involved primarily in cardiovascular control, as well as to the pontine and medullary nuclei controlling respiratory rhythms. The NTS therefore serves a critical integratory function for both circulation and respiration, as described further on.
Perhaps the major advance in our understanding of the autonomic nervous system occurred with the elaboration of the autonomic regulating functions of the hypothalamus. Small, insignificant-appearing nuclei in the walls of the third ventricle and in buried parts of the limbic cortex have rich bidirectional connections with autonomic centers in various parts of the nervous system. As indicated in Chap. 25, the hypothalamus serves as the integrating mechanism of the autonomic nervous system and limbic system. The regulatory activity of the hypothalamus is accomplished in two ways, through direct pathways that descend to particular groups of cells in the brainstem and spinal cord, and through the pituitary and thence to other endocrine glands. The supranuclear regulatory apparatus of the hypothalamus includes three main cerebral structures: the frontal lobe cortex, the insular cortex, and the amygdaloid and adjacent nuclei.
The ventromedial prefrontal and cingulate cortices function as the highest levels of autonomic integration. Stimulation of one frontal lobe may evoke changes in temperature and sweating in the contralateral arm and leg; massive lesions here, which usually cause a hemiplegia, may modify the autonomic functions in the direction of either inhibition or facilitation. Lesions involving the posterior part of the superior frontal and anterior part of the cingulate gyri (usually bilateral, occasionally unilateral) result in loss of voluntary control of the bladder and bowel. Most likely a large contingent of these fibers terminates in the hypothalamus, which, in turn, sends fibers to the brainstem and spinal cord. The descending spinal pathways from the hypothalamus are believed to lie ventromedial to the corticospinal fibers.
The insular cortex receives projections from the NTS, the parabrachial nucleus of the pons, and the lateral hypothalamic nuclei. Direct stimulation of the insula produces cardiac arrhythmias and a number of other alterations in visceral function. The cingulate and hippocampal gyri and their associated subcortical structures (substantia innominata and the amygdaloid, septal, piriform, habenular, and midbrain tegmental nuclei) have been identified as important cerebral autonomic regulatory centers.
Together they have been called the visceral brain (see Chap. 25). Of particular importance in autonomic regulation is the amygdala, the central nucleus of which is a major site of origin of projections to the hypothalamus and brainstem. The anatomy and the effects of stimulation and ablation of the amygdala have been discussed in Chap. 25, in relation to the neurology of emotion.
In addition to the aforementioned central relationships, it should be noted that important interactions between the autonomic nervous system and the endocrine glands occur at a peripheral level. The best-known example is in the adrenal medulla. A similar relationship pertains to the pineal gland, in which norepinephrine (NE) released from postganglionic fibers that end on pineal cells stimulates several enzymes involved in the biosynthesis of melatonin. Similarly, the juxtaglomerular apparatus of the kidney and the islets of Langerhans of the pancreas may function as neuroendocrine transducers insofar as they convert a neural stimulus (in these cases adrenergic) to an endocrine secretion (renin, glucagon, and insulin, respectively). The numerous autonomic–endocrine interactions are elaborated in the next chapter.
Finally, there is the essential role that the hypothalamus plays in the initiation and regulation of autonomic activity, both sympathetic and parasympathetic. Sympathetic responses are most readily obtained by stimulation of the posterior and lateral regions of the hypothalamus, and parasympathetic responses from the anterior regions. The descending sympathetic fibers are largely or totally uncrossed. According to Carmel, fibers from the caudal hypothalamus at first run in the prerubral field, dorsal and slightly rostral to the red nucleus, and then ventral to the ventrolateral thalamic nuclei; then they descend in the lateral tegmentum of the midbrain, pons, and medulla to synapse in the intermediolateral cell column of the spinal cord. In the medulla, the descending sympathetic pathway is located in the posterolateral retroolivary area, where it is frequently involved in lateral medullary infarctions. In the cervical cord, the fibers run in the posterior angle of the anterior horn (Nathan and Smith). According to the latter authors, some of the fibers supplying sudomotor neurons run outside this area but also remain ipsilateral. Jansen and colleagues, by the use of viral vectors in rodents, were able to label certain neurons of the hypothalamus and the ventral medulla that stimulated sympathetic activity in both the stellate ganglion and the adrenal gland. They hypothesized that this dual control underlies the fight-or-flight response, as described in Chap. 25. By contrast, the pathways of descending parasympathetic fibers are not well defined.
Afferent projections from the spinal cord to the hypothalamus have been demonstrated in animals and provide a potential route by which sensation from somatic and possibly visceral structures may influence autonomic responses.
The function of the autonomic nervous system in its regulation of the visceral organs is to a high degree independent of voluntary control and awareness. Furthermore, when the autonomic nerves are interrupted, these organs continue to function (the organism survives), but they are no longer as effective in maintaining homeostasis and adapting to the demands of changing internal conditions and external stresses.
Viscera have a double-nerve supply, sympathetic and parasympathetic and in general these two parts of the autonomic nervous system exert opposite effects. For example, the effects of the sympathetic nervous system on the heart are excitatory and those of the parasympathetic inhibitory. However, some structures—sweat glands, cutaneous blood vessels, and hair follicles—receive only sympathetic postganglionic fibers, and the adrenal gland, as indicated earlier, has only a preganglionic sympathetic innervation. Also, some parasympathetic neurons have been identified in sympathetic ganglia.
All autonomic functions are mediated through the release of chemical transmitters. The modern concept of neurohumoral transmission had its beginnings in the early decades of the twentieth century. In 1921, Loewi discovered that stimulation of the vagus nerve released a chemical substance (Vagusstoff) that slowed the heart. Later this substance was shown by Dale to be acetylcholine (ACh). Also, in 1920, Cannon reported that stimulation of the sympathetic trunk released an epinephrine-like substance, which increased the heart rate and blood pressure. He named this substance “sympathin,” subsequently shown to be noradrenaline, or NE. Dale found that ACh had pharmacologic effects similar to those obtained by stimulation of parasympathetic nerves; he designated these effects as “parasympathomimetic.” These observations placed neurochemical transmission on solid ground and laid the basis for the distinction between cholinergic and adrenergic transmission in the autonomic nervous system.
The most important of the autonomic neurotransmitters are ACh and NE. ACh is synthesized at the terminals of axons and stored in presynaptic vesicles until it is released by the arrival of nerve impulses. ACh is released at the terminals of all preganglionic fibers (in both the sympathetic and parasympathetic ganglia), as well as at the terminals of all postganglionic parasympathetic and a few special postganglionic sympathetic fibers, mainly those subserving sweat glands. Of course, ACh is also the chemical transmitter of nerve impulses to the skeletal muscle fibers. Parasympathetic postganglionic function is mediated by two distinct types of ACh receptors: nicotinic and muscarinic, so named by Dale because the choline-induced responses were similar either to those of nicotine or to those of the alkaloid, muscarine. The postganglionic parasympathetic receptors are located within the innervated organ and are muscarinic; i.e., they are antagonized by atropinic drugs. As already mentioned the receptors in ganglia, like those of skeletal muscle, are nicotinic; they are not blocked by atropine but are counteracted by other agents (e.g., tubocurarine).
It is likely that more than ACh is involved in nerve transmission at a ganglionic level. Many peptides—substance P, enkephalins, somatostatin, vasoactive intestinal peptide, adenosine triphosphate (ATP), and nitric oxide—have been identified in the autonomic ganglia, localizing in some cases to the same cell as ACh. (This negates “Dale’s principle,” or “law,” which stipulates that one neuron elaborates only one neurotransmitter.) Particular neuronal firing rates appear to cause the preferential release of one or another of these substances. Most of the neuropeptides exert their postsynaptic effects through the G-protein transduction system, which uses adenyl cyclase or phospholipase C as an intermediary. The neuropeptides act as modulators of neural transmission, although their exact function in many cases remains to be determined.
With two exceptions, postganglionic sympathetic fibers release only NE at their terminals. The sweat glands and some blood vessels in muscle are innervated by postganglionic sympathetic fibers, but their terminals, as mentioned, release ACh. The NE that is discharged into the synaptic space activates specific adrenergic receptors on the postsynaptic membrane of target cells.
Adrenergic receptors are of two types, classified originally by Ahlquist as alpha and beta. In general, the alpha receptors mediate vasoconstriction, relaxation of the gut, and dilatation of the pupil; beta receptors mediate vasodilatation, especially in muscles, relaxation of the bronchi, and an increased rate and contractility of the heart. Each of these receptors is subdivided further into two types. Alpha1 receptors are postsynaptic; alpha2 receptors are situated on the presynaptic membrane and, when stimulated, diminish the release of the transmitter. Beta1 receptors are, for all practical purposes, limited to the heart; their activation increases the heart rate and contractility. Beta2 receptors, when stimulated, relax the smooth muscle of the bronchi and of most other sites, including the blood vessels of skeletal muscle. A comprehensive account of neurohumoral transmission and receptor function can be found in the monograph by Cooper and colleagues.
Discussed in the following pages are the ways in which the two divisions of the autonomic nervous system, acting in conjunction with the endocrine glands, maintain the homeostasis of the organism. As stated earlier, the integration of these two systems is achieved primarily in the hypothalamus. In addition, the endocrine glands are influenced by circulating catecholamines, and some of them are innervated by adrenergic fibers. Chapter 27 discusses further these autonomic–endocrine relations.
As was indicated briefly in Chap. 18, blood pressure depends on the adequacy of intravascular blood volume, on systemic vascular resistance, and on the cardiac output. Both the autonomic and endocrine systems influence the muscular, cutaneous, and mesenteric (splanchnic) vascular beds, heart rate, and stroke volume of the heart. Together, these actions serve to maintain normal blood pressure and allow reflex maintenance of blood pressure with changes in body position. Two types of baroreceptors function as the afferent component of this reflex arc by sensing pressure gradients across the walls of large blood vessels. Those in the carotid sinus and aortic arch are sensitive to reductions in pulse pressure (the difference between systolic and diastolic blood pressure), while those in the right heart chambers and pulmonary vessels respond more to alterations in blood volume. The carotid sinus baroreceptors are rapidly responsive and capable of detecting beat-to-beat changes, in contrast to the aortic arch nerves, which have a longer response time and discriminate only the larger and more prolonged alterations in pressure.
The nerves arising from these receptors are small-caliber, thinly myelinated fibers that course in cranial nerves IX and X and terminate in the nucleus of the tractus solitarius (NTS). In response to increased stimulation of these receptors, vagal efferent activity is reduced, resulting in reflex cardioacceleration. This is accomplished through polysynaptic connections between the NTS and the dorsal motor nucleus of the vagus; it is from this structure that vagal neurons project to the sinoatrial node, atrioventricular node, and the muscle of the left ventricle. Thus, vagal activity results in reduction in heart rate and in the contractile force of the myocardium (negative inotropy). Increased systemic vascular resistance is mediated concurrently through parallel connections between the NTS and the medullary pressor areas that project to the intermediolateral cells of the midthoracic cord. The main sympathetic outflow from these thoracic segments is via the greater splanchnic nerve to the celiac ganglion, the postganglionic nerves of which project to the capacitance vessels of the gut. The splanchnic capacitance veins act as a reservoir for as much as 20 percent of the total blood volume, and interruption of the splanchnic nerves results in severe postural hypotension. After a high-carbohydrate meal there is a marked hyperemia of the gut and compensatory peripheral vasoconstriction in the muscles and skin. It has also been noted that the mesenteric vascular bed is responsive to the orthostatic redistribution of blood volume but not to mental stress.
The opposite response to the one described earlier, namely bradycardia and hypotension, results when vagal tone is enhanced and sympathetic tone reduced. This response can be triggered by baroreceptors, or it may arise from cerebral stimuli such as fear or sight of blood in susceptible individuals as well as from extreme pain, particularly arising in the viscera.
Two slower-acting humoral mechanisms regulate blood volume and complement the control of systemic vascular resistance. Pressure-sensitive renal juxtaglomerular cells release renin, which stimulates production of angiotensin and influences aldosterone production, both of which affect an increase of blood volume. Of lesser influence in the control of blood pressure is antidiuretic hormone, discussed in the next chapter; but the effects of this peptide become more important when autonomic failure forces a dependence on secondary mechanisms for the maintenance of blood pressure. In addition to its presence in autonomic ganglia, nitric oxide has been found to have an important local role in maintaining vascular tone, mainly by way of attenuating the response to sympathetic stimulation. The extent to which this latter function is under neural control is not clear.
The familiar functions of the bladder and lower urinary tract—the storage and intermittent evacuation of urine—are served by three structural components: the bladder itself, the main component of which is the large detrusor (transitional type) muscle; a functional internal sphincter composed of similar muscle; and the striated external sphincter or urogenital diaphragm. The sphincters assure continence; in the male, the internal sphincter also prevents the reflux of semen from the urethra during ejaculation. For micturition to occur, the sphincters must relax, allowing the detrusor to expel urine from the bladder into the urethra. This is accomplished by a complex mechanism involving mainly the parasympathetic nervous system (the sacral peripheral nerves derived from the second, third, and fourth sacral segments of the spinal cord and their somatic sensorimotor fibers) and, to a lesser extent, sympathetic fibers derived from the thorax. The vaguely localizable brainstem “micturition centers,” with their spinal and suprasegmental connections, may contribute (Fig. 26-4).
The detrusor muscle receives motor innervation from nerve cells in the intermediolateral columns of gray matter, mainly from the third and also from the second and fourth sacral segments of the spinal cord (the “detrusor center”). These neurons give rise to preganglionic fibers that synapse in parasympathetic ganglia within the bladder wall. Short postganglionic fibers end on muscarinic acetylcholine receptors of the muscle fibers. There are also beta-adrenergic receptors in the dome of the bladder, which are activated by sympathetic fibers that arise in the intermediolateral nerve cells of T10, T11, and T12 segments. These preganglionic fibers pass via inferior splanchnic nerves to the inferior mesenteric ganglia (see Fig. 26-1); pre- and postganglionic sympathetic axons are conveyed by the hypogastric nerve to the pelvic plexus and the bladder dome. The internal sphincter and base of the bladder (trigone), consisting of smooth muscle, are also innervated to some extent by the sympathetic fibers of the hypogastric nerves; their receptors are mainly of alpha-adrenergic type, which makes it possible to therapeutically manipulate the function of the sphincter with adrenergically active drugs as well as the more commonly used cholinergic ones (see further on).
The external urethral and anal sphincters are composed of striated muscle fibers. Their innervation, via the pudendal nerves, is derived from a densely packed group of somatomotor neurons (nucleus of Onuf) in the anterolateral horns of sacral segments 2, 3, and 4. Cells in the ventrolateral part of Onuf’s nucleus innervate the external urethral sphincter, and cells of the mediodorsal part innervate the anal sphincter. The muscle fibers of the sphincters respond to the nicotinic effects of ACh.
The pudendal nerves also contain afferent fibers coursing from the urethra and the external sphincter to the sacral segments of the spinal cord. These fibers convey impulses for reflex activities and, through connections with higher centers, for sensation. Some of these fibers probably course through the hypogastric plexus, as indicated by the fact that patients with complete transverse lesions of the cord as high as T12 may report vague sensations of urethral discomfort. The bladder is sensitive to pain and pressure; these senses are transmitted to higher centers along the sensory pathways described in Chaps. 8 and 9.
Unlike skeletal striated muscle, the detrusor, because of its postganglionic system, is capable of some contractions, although imperfect, after complete destruction of the sacral segments of the spinal cord. Isolation of the sacral cord centers (transverse lesions of the cord above the sacral levels) and their peripheral nerves permits contractions of the detrusor muscle, but they still do not empty the bladder completely; patients with such lesions usually develop dyssynergia of the detrusor and external sphincter muscles (see later), indicating that coordination of these muscles must occur at supraspinal levels (Blaivas). With acute transverse lesions of the upper cord, the function of sacral segments is abolished for several weeks in the same way as the motor neurons of skeletal muscles (the state of spinal shock).
The storage of urine and the efficient emptying of the bladder are possible only when the spinal segments, together with their afferent and efferent nerve fibers, are connected with the so-called micturition centers in the pontomesencephalic tegmentum. In experimental animals, this center (or centers) lies within or adjacent to the locus ceruleus. A medial region triggers micturition, while a lateral area seems more important for continence. These neurons receive afferent impulses from the sacral cord segments; their efferent fibers course downward via the reticulospinal tracts in the lateral funiculi of the spinal cord and activate cells in the nucleus of Onuf, as well as in the intermediolateral cell groups of the sacral segments (Holstege and Tan). In cats, the pontomesencephalic centers receive descending fibers from anteromedial parts of the frontal cortex, thalamus, hypothalamus, and cerebellum, but the brainstem centers and their descending pathways have not been precisely defined in humans. Other fibers from the motor cortex descend with the corticospinal fibers to the anterior horn cells of the sacral cord and innervate the external sphincter. According to Ruch, the descending pathways from the midbrain tegmentum are inhibitory and those from the pontine tegmentum and posterior hypothalamus are facilitatory. The pathway that descends with the corticospinal tract from the motor cortex is inhibitory. Thus the net effect of lesions in the brain and spinal cord on the micturition reflex, at least in animals, may be either inhibitory or facilitatory (DeGroat).
Almost all of this information has been inferred from animal experiments; there is little human pathologic material to corroborate the role of brainstem nuclei and cortex in bladder control. What information is available is reviewed extensively by Fowler, whose article is recommended. Also of interest here is the study by Blok and colleagues, who performed positron emission tomography (PET) studies in volunteer subjects during micturition. Increased blood flow was detected in the right pontine tegmentum, periaqueductal region, hypothalamus, and right inferior frontal cortex. When the bladder was full but subjects were prevented from voiding, increased activity was seen in the right ventral pontine tegmentum. The meaning of these lateralized findings is unclear, but the study supports the presumption that pontine centers are involved in the act of voiding.
The act of micturition is both reflex and voluntary. When the normal person desires to void, there is first a voluntary relaxation of the perineum, followed sequentially by an increased tension of the abdominal wall, a slow contraction of the detrusor, and an associated opening of the internal sphincter; finally, there is a relaxation of the external sphincter (Denny-Brown and Robertson). It is useful to think of the detrusor contraction as a spinal stretch reflex, subject to facilitation and inhibition from higher centers. Voluntary closure of the external sphincter and contraction of the perineal muscles cause the detrusor contraction to subside. The abdominal muscles have little role in initiating micturition except when the detrusor muscle is not functioning normally. The voluntary restraint of micturition is a cerebral affair and is mediated by fibers that arise in the frontal lobes (paracentral motor region), descend in the spinal cord just anterior and medial to the corticospinal tracts, and terminate on the cells of the anterior horns and intermediolateral cell columns of the sacral segments, as described earlier. The coordination of detrusor and external sphincteric function depends mainly on the descending pathway from the posited centers in the dorsolateral pontine tegmentum.
The colon and anal sphincters are obedient to the same principles that govern bladder function. Unique to the bowel, however, is an intrinsic enteric nervous system that originates in the myenteric (or Auerbach) plexus and the submucosal plexus (of Meissner), located in the gut wall. The first stimulates smooth muscle and the latter also regulates mucosal secretion and blood flow. This embedded system controls peristalsis largely independent of other autonomic influences but is highly responsive to local chemical and mechanical stimuli. As outlined in the thorough review by Benarroch that should be consulted by interested readers, acetylcholine is the dominant neurotransmitter in the enteric nerves but nitric oxide and numerous peptide transmitters are found in profusion.
Inasmuch as the autonomic nervous system and the adrenal glands were accepted for many years as the neural and humoral basis of all instinctive and emotional behavior. In states of chronic anxiety and acute panic reactions, depressive psychosis, mania, and schizophrenia, all of which are characterized by an altered emotionality, no consistent autonomic or endocrine dysfunction has been demonstrated except perhaps for diminished responses of growth hormone in panic disorders. The lack of cortisol suppressibilty by injection of adrenocorticotropic hormone (ACTH) had for some time also been considered to be a consistent aspect of depressive illnesses but that too, has not been entirely specific. This has been disappointing, as the emergency theory of sympathoadrenal action provided by Cannon was such a promising concept of the neurophysiology of acute emotion, and Selye had extended this theory so plausibly to explain all the reactions to stress in animals and humans.
According to these theories, strong emotion, such as anger or fear, excites the sympathetic nervous system and the adrenal cortex (via corticotropin-releasing factor [CRF] and ACTH), which are under direct neural and endocrine control. These sympathoadrenal reactions are brief and sustain the animal in “flight or fight” as discussed in Chap. 25. Animals deprived of adrenal cortex or human beings with Addison disease cannot tolerate stress because they are incapable of mobilizing both the adrenal medulla and adrenal cortex. Prolonged stress and production of ACTH activates all the adrenal hormones (glucocorticoids, mineralocorticoids, and adrenocorticoids) and has been studied extensively in relation to immune reactions and other systemic functions but with no consistent findings that are yet clinically applicable.
With few exceptions, such as testing pupillary reactions and examination of the skin for abnormalities of color and sweating, the neurologist tends to be casual in evaluating the function of the autonomic nervous system. Nonetheless, several simple but informative tests can be used to confirm one’s clinical impressions and to elicit abnormalities of autonomic function that may aid in diagnosis. For the detection of certain disease, it is almost imperative that blood pressure be evaluated to detect a drop with change in body position from lying or sitting, to standing. A combination of tests is usually necessary, because certain ones are particularly sensitive to abnormalities of sympathetic function and others to parasympathetic or baroreceptor afferent function. These are described later and are summarized in Table 26-1. A scheme for the examination of pupillary abnormalities was presented in Fig. 14-11.
TEST | NORMAL RESPONSE | MAIN PART OF REFLEX ARC TESTED |
---|---|---|
Noninvasive Bedside Tests | ||
Blood-pressure response to standing or vertical tilt | Fall in BP ≤30/15 mm Hg | Afferent and sympathetic efferent limbs |
Heart rate response to standing | Increase 11–90 beats/min; 30:15 ratio ≥1.04 | Vagal afferent and efferent limbs |
Isometric exercise | Increase in diastolic BP, 15 mm Hg | Sympathetic efferent limb |
Heart rate variation with respiration | Maximum-minimum heart rate ≥15 beats/min; E:I ratio 1.2a | Vagal afferent and efferent limbs |
Valsalva ratio (see text) | ≥1.4a | Afferent and efferent limbs |
Sweat tests | Sweating over all body and limbs | Sympathetic efferent limb |
Axon reflex | Local piloerection, sweating | Postganglionic sympathetic efferent fibers |
Plasma noradrenaline level | Rises on tilting from horizontal to vertical | Sympathetic efferent limb |
Plasma vasopressin level | Rise with induced hypotension | Afferent limb |
Invasive Tests | ||
Valsalva maneuver (BP response with indwelling arterial catheter or continuous noninvasive BP measurement) | Phase I: Rise in BP | Afferent and sympathetic efferent limbs |
Phase II: Gradual reduction of BP to plateau; tachycardia | ||
Phase III: Fall in BP | ||
Phase IV: Overshoot of BP, bradycardiaa | ||
Baroreflex sensitivity | (1) Slowing of heart rate with induced rise of BPa | (1) Parasympathetic afferent and efferent limbs |
(2) Steady-state responses to induced rise and fall of BP | (2) Afferent and efferent limbs | |
Infusion of pressor drugs | (1) Rise in BP | (1) Adrenergic receptors |
(2) Slowing of heart rate | (2) Afferent and efferent parasympathetic limbs | |
Other Tests of Vasomotor Control | ||
Radiant heating of trunk | Increased hand blood flow | Sympathetic efferent limb |
Immersion of hand in hot water | Increased blood flow of opposite hand | Sympathetic efferent limb |
Cold pressor test | Reduced blood flow, rise in BP | Sympathetic efferent limb |
Emotional stress | Increased BP | Sympathetic efferent limb |
Tests of Pupillary Innervation | ||
4% cocaine | Pupil dilates | Sympathetic innervation |
0.1% adrenaline | No response | Postganglionic sympathetic innervation |
1% hydroxyamphetamine hydrobromide | Pupil dilates | Postganglionic sympathetic innervation |
2.5% methacholine 0.125% pilocarpine | No response | Parasympathetic innervation |
0.5% apraclonidine | Pupil dilates and ptosis resolves in Horner syndrome | Parasympathetic innervation |
These are among the simplest and most important tests of autonomic function and most laboratories have automated techniques to quantitate them. McLeod and Tuck state that in changing from the recumbent to the standing position, a fall of more than 30 mm Hg systolic and 15 mm Hg diastolic is abnormal; others give figures of 20 and 10 mm Hg. They caution that the arm on which the cuff is placed must be held horizontally when standing, so that the decline in arm pressure will not be obscured by the added hydrostatic pressure. As emphasized in Chap. 18 on syncope, the determination of blood pressure in orthostatic testing is ideally done by having the patient remain supine for as long as it is practical before testing, and shifting from a supine to standing position, without the interposition of sitting. Moreover, blood pressure is most informative if measured immediately after standing and again at approximately 1 and 3 min. The expected response is a momentary and slight increase in pressure that is usually not detected with a manual blood pressure cuff, followed by a slight drop within seconds of standing, and then a slow recovery during the first minute. Persistent hypotension at 1 min indicates sympathetic adrenergic failure and the later measurement affirms this if blood pressure fails to recover or continues to decline (Fig. 26-5A).
Figure 26-5.
A. Blood pressure and heart rate changes elicited by standing and head-up tilt in a healthy individual. B. Blood pressure and heart rate changes elicited by Valsalva in a healthy individual. (With permission from Bannister R: Autonomic Failure: A Textbook of Clinical Disorders of the Autonomic Nervous System, 2nd ed. Oxford, Oxford University Press, 1988.)
The main cause of an orthostatic drop in blood pressure is, of course, hypovolemia. In the context of recurrent fainting, however, an excessive drop reflects inadequate sympathetic vasoconstrictor activity. The use of a tilt table, as described in “Tilt-Table Testing” in Chap. 18 and further on, is an additional means of inducing orthostatic changes and also elicits reflex fainting in patients prone to syncope from an oversensitive cardiac reflex, i.e., one that produces vasodilatation (neurocardiogenic syncope). In response to the induced drop in blood pressure, the heart rate (under vagal control) normally increases. The failure of the heart rate to rise in response to the drop in blood pressure with standing is the simplest bedside indicator of vagal nerve dysfunction. Neurally mediated syncope may show one of three initial patterns with testing on a tilt table: a paroxysmal vasodepressor response alone, a combined bradycardic and hypotensive response, and solely bradycardia. The tilt table allows differentiation among these or, more often, clarifies the order in which the events occur.
In addition, the heart rate, after rising initially in response to upright standing posture (not with the tilt table), slows after about 15 beats to reach a stable rate by the thirtieth beat. The ratio of R-R intervals in the electrocardiogram (ECG), corresponding to the thirtieth and fifteenth beats (the 30:15 ratio), is an even more sensitive measure of the integrity of vagal inhibition of the sinus node. A ratio in adults under age 60 of less than 1.07 is usually abnormal, indicating a loss of vagal tone and the normal ratio is progressively higher for younger ages, for example, it is usually above 1.12 at age 30 and 1.1 at age 40.
Another simple procedure for quantitating purely vagal function consists of measuring the variation in heart rate during deep breathing (respiratory sinus arrhythmia). The ECG is recorded while the patient breathes at a regular rate of 6 breaths per minute. Normally, the heart rate varies by as many as 15 beats per minute or even more between expiration and inspiration; differences of less than 7 beats per minute for ages 60 to 69 and 9 for ages 50 to 59 may be abnormal.
A yet more accurate test of vagal function is the measurement of the ratio of the longest R-R interval during forceful slow expiration (standardized as constant blowing at a pressure of 40 mm Hg for 10 s) to the shortest R-R interval during inspiration, which allows the derivation of an expiration–inspiration (E:I) ratio. This is the best validated of all the heart-rate measurements, particularly as computerized methods can be used to display the spectrum of beat-to-beat ECG intervals during breathing. The results of these tests must always be compared with those obtained in normal individuals of the same age. Up to age 40 years, E:I ratios of less than 1.2 (signifying a variation of 20 percent) are abnormal. The ratio decreases with age, and markedly so beyond age 60 years (at which time it approaches 1.04 or less), as it does also in the presence of even mild diabetic neuropathy. Thus the test results must be interpreted cautiously in the elderly or in diabetic individuals. Similar ratios have been developed for heart-rate change during the Valsalva maneuver; the Valsalva ratio.
Computerized methods of power spectral analysis may be used to express the variance in heart rate as a function of the beat-to-beat interval. Several power peaks are appreciated: one related to the respiratory sinus arrhythmia and others that reflect baroreceptor and cardiac sympathetic activity. All of these tests of heart-rate variation are usually combined with measurement of heart rate and blood pressure during the Valsalva maneuver, as described below, and with the tilt-table test, as described in Chap. 18.
In the Valsalva maneuver, the subject exhales into a manometer or against a closed glottis for 10 to 15 s, creating a markedly positive intrathoracic pressure. The sharp reduction in venous return to the heart causes a drop in cardiac output and in blood pressure; the response on baroreceptors is to cause a reflex tachycardia and, to a lesser extent, peripheral vasoconstriction. With release of intrathoracic pressure, the venous return, stroke volume, and blood pressure rise to higher-than-normal levels; reflex parasympathetic influence then predominates and a bradycardia results (Fig. 26-5B). Failure of the heart rate to increase during the positive intrathoracic pressure phase of the Valsalva maneuver points to sympathetic dysfunction, and failure of the rate to slow during the period of blood pressure overshoot points to a parasympathetic disturbance. In patients with autonomic failure, the fall in blood pressure is not aborted during the last few seconds of increased intrathoracic pressure, and there is no overshoot of blood pressure when the breath is released. The Valsalva ratio, referring to the maximum heart rate generated by the maneuver to the lowest heart rate within 30 s of that peak, is another often-used measure in comprehensive autonomic testing.
These generally test sympathetic cholinergic function. Measurement of the skin temperature is a rough but useful index of vasomotor function. Vasomotor paralysis results in vasodilatation of skin vessels and a rise in skin temperature; vasoconstriction lowers the temperature. With a skin thermometer, one may compare affected and normal areas under standard conditions. The normal skin temperature is 31°C (87.8°F) to 33°C (91.4°F) when the room temperature is 26°C (78.8°F) to 27°C (80.6°F). Vasoconstrictor tone may also be tested by measuring the reduction in skin temperature at a distant site before and after immersing one or both hands in cold water (see the discussion of the cold pressor test later).
The integrity of the sympathetic reflex arc, which includes baroreceptors in the aorta and carotid sinus, their afferent pathways, the vasomotor centers, and the sympathetic and parasympathetic outflow can be tested in a general way by combining the cold pressor test, grip test, mental arithmetic test, and Valsalva maneuver, as described below.
Vasoconstriction induces an elevation of the blood pressure. This is the basis of the cold pressor test. In normal persons, immersing one hand in ice water for 1 to 5 min raises the systolic pressure by 15 to 20 mm Hg and the diastolic pressure by 10 to 15 mm Hg. Similarly, the sustained isometric contraction of a group of muscles (e.g., those of the forearm in handgrip) for 5 min normally increases the heart rate and the systolic and diastolic pressures by at least 15 mm Hg. The response in both of these tests is reduced or absent with lesions of the sympathetic reflex arc, particularly of the efferent limb, but neither of these tests has been well quantitated or validated. The stress involved in doing mental arithmetic in noisy and distracting surroundings will also stimulate a mild but measurable increase in pulse rate and blood pressure. Obviously this response does not depend on the afferent limb of the sympathetic reflex arc and must be mediated by cortical–hypothalamic mechanisms.
If the response to the Valsalva maneuver is abnormal and the response to the cold pressor test is normal, the lesion is probably in the baroreceptors or their afferent nerves; such a defect has been found in diabetic and tabetic patients and is common in many neuropathies. A failure of the heart rate and blood pressure to rise during mental arithmetic coupled with an abnormal Valsalva maneuver suggests a defect in the central or peripheral efferent sympathetic pathways.
The integrity of sympathetic efferent pathways can be assessed further by tests of sudomotor activity. There are several of these, all used mainly in specialized autonomic testing laboratories; furthermore, most of them cannot differentiate central from peripheral causes of anhidrosis. The most rudimentary tests involve weighing sweat after it is absorbed by small squares of filter paper. Also, powdered charcoal dusted on the skin will cling to moist areas and not to dry ones.
In the sympathetic or galvanic skin-resistance test, a set of electrodes placed on the skin measures the resistance to the passage of a weak current through the skin; in all likelihood, the change in electrical potential is the result of an ionic current within the sweat glands, not simply an increase in sweating that lowers skin resistance. This method can be used to outline an area of reduced sweating because of a peripheral nerve lesion, as the response depends on sympathetic activation of sweat glands (Gutrecht). However, the galvanic skin response is subject to habituation with repeated stimuli and will show no response if there is a sensory neuropathy. The starch iodine test or use of a color indicator such as quinizarin (gray when dry, purple when wet) and the more recently introduced plastic or silicone method are other acceptable procedures to delineate peripheral nerve or spinal cord lesions based on the loss of sympathetic innervation. Together they are termed “thermoregulatory sweat testing” and are semiquantitave and mainly topographic in nature. They reflect postganglionic sudomotor function.
A more quantitative and reproducible examination of postganglionic sudomotor function, termed QSART (quantitative sudomotor axon reflex test), has been developed and studied extensively by Low. It is essentially a test of distal sympathetic axonal integrity utilizing the local axon reflex. A 10 percent solution of acetylcholine is iontophoresed onto the skin using 2 mA for 5 min. Sweat output is recorded in the adjacent skin by sophisticated circular cells that detect the sweat water. The forearm, proximal leg, distal leg, and foot have been chosen as standardized recording sites. By this test, Low has been able to define patterns of absent or delayed sweating that signify postganglionic sympathetic failure in small-fiber neuropathies and excessive sweating or reduced latency in response, as is seen in reflex sympathetic dystrophy. This is the preferred method of studying sweating and the function of distal sympathetic fibers, but its technical complexity makes it available only in specially equipped laboratories.
Tearing can be estimated roughly by inserting one end of a 5-mm-wide and 25-mm-long strip of thin filter paper into the lower conjunctival sac while the other end hangs over the edge of the lower lid (the Schirmer test). The tears wet the strip of filter paper, producing a moisture front. After 5 min, the moistened area extends for a length of approximately 15 mm in normal persons. An extent of less than 10 mm is suggestive of hypolacrima. This test is used mainly to detect the dry eyes (keratoconjunctivitis sicca) of the Sjögren syndrome, but it may also be helpful in fully studying various autonomic neuropathies.
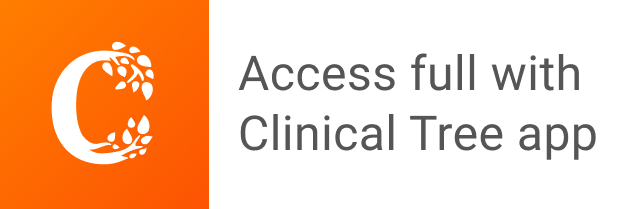