Disturbances of Cerebrospinal Fluid, Including Hydrocephalus, Pseudotumor Cerebri, and Low-Pressure Syndromes: Introduction
In the chapters to follow, reference is made to the ways in which changes in the cerebrospinal fluid (CSF) reflect the basic pathologic processes in a variety of inflammatory and infectious, neoplastic, demyelinative, and degenerative diseases. The CSF alterations in these circumstances raise so many important problems that we consider it worthwhile to discuss in one place the mechanisms involved in the formation, circulation, and absorption of the CSF, particularly as they pertain to alterations of intracranial pressure (ICP). Considered in this chapter are hydrocephalus, pseudotumor cerebri, and syndromes produced by reduced pressure in the CSF compartment. Further information on the management of raised ICP as it pertains to traumatic brain injury, of which the CSF is an essential part, can be found in Chap. 35. Examination of the CSF as a diagnostic aid in neurology was discussed in Chap. 2, and the primary infectious and noninfectious inflammatory reactions of the pia-arachnoid (leptomeninges) and ependyma of the ventricles are considered in Chap. 32.
A few historical points call to mind that our understanding of the physiology, chemistry, and cytology of the CSF is the result of a technical innovation that was introduced a century ago. Although the lumbar puncture was introduced by Quincke in 1891, it was not until 1912 that Mestrezat made correlations between disease processes and the cellular and chemical changes in the CSF. In 1937, Merritt and Fremont-Smith published their monograph on CSF changes in a broad variety of disease. Our knowledge of CSF cytology has accumulated since the late 1950s, when membrane filtration techniques (particularly the cellulose ester or Millipore filter) were introduced. The studies of Dandy (1919) and of Weed (1935) provided the basis of our knowledge of CSF formation, circulation, and absorption. The important studies of Pappenheimer and of Ames and their colleagues followed, and then the monographs of Fishman and of Davson and coworkers, which are important modern contributions. (See Chap. 2 for references.) Recent inceptions in analyzing the lymphocytic cells and protein fractions in the CSF have expanded on modern technology that was developed in hematology.
Physiology of the Cerebrospinal Fluid
The primary function of the CSF appears to be a mechanical one; it serves as a kind of water jacket for the spinal cord and brain, protecting them from potentially injurious blows to the spinal column and skull and acute changes in venous pressure. Also, it provides the brain with buoyancy. As pointed out by Fishman, the 1,500-g brain, which has a water content of approximately 80 percent, weighs only 50 g when suspended in CSF, so the brain virtually floats in its CSF. Many of the physiologic mechanisms described below are committed to maintaining the relatively constant volume–pressure relationships of the CSF. In addition, because the brain and spinal cord have no lymphatic channels, the CSF, through its “sink action,” serves to remove waste products of cerebral metabolism, the main ones being CO2, lactate, and hydrogen ions. The composition of the CSF is maintained within narrow limits, despite major alterations in the blood; thus the other main function of CSF, along with the intercellular fluid of the brain, is to preserve a stable chemical environment for neurons, astrocytes, and myelinated fibers. There is no reason, however, to believe that the CSF is actively involved in the metabolism of the cells of the brain, and spinal cord.
In the adult, the average intracranial volume is 1,700 mL; the volume of the brain is from 1,200 to 1,400 mL, CSF volume ranges from 70 to 160 mL (mean: 104 mL), and that of blood is approximately 150 mL. In addition, the spinal subarachnoid space contains 10 to 25 mL of CSF. Thus, at most, the CSF occupies somewhat less than 10 percent of the intracranial and intraspinal spaces. The proportion of CSF in the ventricles, and cerebral cisternae and sulci in the subarachnoid spaces varies with age. These variations have been plotted in CT scans by Meese and coworkers; the distance between the caudate nuclei at the anterior horns gradually widens by approximately 1.0 to 1.5 cm, and the width of the third ventricle increases from 3 to 6 mm by age 60 years.
The introduction of the ventriculocisternal perfusion technique by Pappenheimer and colleagues made possible measurements of the rates of formation and absorption of the CSF. They established that the average rate of CSF formation is 21 to 22 mL/h (0.35 mL/min), or approximately 500 mL/d; thus the volume of CSF as a whole is renewed 4 or 5 times daily.
The choroid plexuses, located in the floor of the lateral, third, and fourth ventricles, are the main sites of CSF formation (some CSF is formed by the meninges even after the choroid plexuses are removed). The thin-walled vessels of the plexuses allow passive diffusion of substances from the blood plasma into the extracellular space surrounding choroid cells. The choroidal epithelial cells, like other secretory epithelia, contain organelles, indicating their capacity for an energy-dependent secretory function, i.e., “active transport.” The blood vessels in the subependymal regions and the pia also contribute to the CSF, and some substances enter the CSF as readily from the meninges as from the choroid plexuses. Thus, electrolytes equilibrate with the CSF at all points in the ventricular and subarachnoid spaces, and the same is true of glucose. The transport of sodium, the main cation of the CSF, is accomplished by the action of a sodium-potassium-ion exchange pump at the apical surface of the choroid plexus cells, the energy for which is supplied by adenosine tri-phosphate (ATP); drugs that inhibit the ATP system therefore reduce CSF formation (Cutler and Spertell). Electrolytes enter the ventricles somewhat more readily than they enter the subarachnoid space (water does the opposite). It is also known that the penetration of certain drugs and metabolites into the CSF (and brain) is in direct relation to their lipid solubility. Ionized compounds, such as hexoses and amino acids, being relatively insoluble in lipids, enter the CSF slowly unless facilitated by a membrane transport system. This type of facilitated (carrier) diffusion is stereospecific; i.e., the carrier (a specific protein or proteolipid) binds only with a solute having a specific configuration, and conducts it across the membrane, where it is released into the CSF, and intercellular fluid.
Diffusion gradients appear to determine the entry of serum electrolytes and proteins into the CSF, and also the exchanges of CO2. Water and sodium diffuse as readily from blood to CSF and intercellular spaces as in the reverse direction. This explains the rapid effects of intravenously injected hypotonic and hypertonic fluids. Studies using radioisotopic tracer techniques show that the various constituents of the CSF (see Table 2-2) are in dynamic equilibrium with the blood. Similarly, CSF in the ventricles and subarachnoid spaces is in equilibrium with the intercellular fluid of the brain, spinal cord, and olfactory and optic nerves. Certain structures that maintain this equilibrium are subsumed under the term blood–brain barrier, which is used to designate all of the interfaces between blood, brain, and CSF. The site of the barrier varies for the different plasma constituents. One is the endothelium of the choroidal and brain capillaries; another is the plasma membrane and adventitia (Rouget cells) of these vessels; a third is the pericapillary foot processes of astrocytes. Large molecules, such as albumin, are prevented from entry by the capillary endothelium, and this is the barrier also for such molecules as are bound to albumin, e.g., aniline dyes (trypan blue), bilirubin, and many drugs. Other smaller molecules are blocked from entering the brain by the capillary plasma membrane or astrocytes.
The substances formed in the nervous system during its metabolic activity diffuse rapidly into the CSF. Thus, the CSF has, as mentioned, a “sink action,” to use Davson’s term, by which the products of brain metabolism are removed into the bloodstream as CSF is absorbed.
Harvey Cushing aptly termed the CSF the “third circulation,” comparable to that of blood and lymph. From its principal site of formation in the choroid plexus of the lateral ventricles, CSF flows downward sequentially through the third ventricle, aqueduct, fourth ventricle, and foramina of Magendie (medially) and Luschka (laterally) at the base of the medulla, to the perimedullary and perispinal subarachnoid spaces, thence around the brainstem and rostrally to the basal and ambient cisterns, through the tentorial aperture, and finally to the lateral and superior surfaces of the cerebral hemispheres, where most of it is absorbed (Fig. 30-1). The pressure in the CSF compartment is highest in the ventricles and diminishes successively along the subarachnoid pathways. Arterial pulsations of the choroid plexuses help drive the fluid centrifugally from the ventricular system.
Figure 30-1.
A. Schematic representation of the three components of the intracranial contents: the incompressible brain tissue (orange); the vascular system (dark blue channel); and the CSF (light blue). The red outer circle represents the intracranial compartment, which is fixed in size. B. With ventricular outflow obstruction. C. With obstruction at or near the points of outlet of the CSF. D. With obstruction of the venous outflow. (Redrawn with permission from Foley.)
The spinal fluid is in contact everywhere with the extracellular fluid of the brain and spinal cord, but the extent of bulk flow of the CSF through the brain parenchyma is small under normal conditions. The periventricular tissue offer considerable resistance to the entrance of CSF and although the pressure difference between the ventricles and the subarachnoid spaces over the convexity of the hemispheres (transmantle pressure) is above zero, the open ventricular–foraminal–subarachnoid pathway directs the bulk of CSF flow in this direction. Only if this conduit is obstructed does the transmantle pressure rise, compressing the periventricular tissues and leading to ventricular enlargement, i.e., hydrocephalus, and transependymal flow of CSF.
Absorption of CSF is mainly through the arachnoid villi. These are microscopic excrescences of arachnoid membrane that penetrate the dura and protrude into the superior sagittal sinus and other venous structures. Multiple villi are aggregated in these locations to form the pacchionian granulations or bodies, some of them large enough to indent the inner table of the calvarium. These may increase in size with aging, and large ones (3.0 to 9.5 mm in diameter) may rarely herniate through bony defects and erode, providing a portal of entry to organisms from the ear that lead to meningitis (Samuels et al).
The arachnoid villi, most numerous on both sides of the superior sagittal sinus, are also present at the base of the brain and around the spinal cord roots and have been thought to act as functional valves that permit unidirectional “bulk flow” of CSF into the vascular lumen. However, electron microscope studies show that the arachnoid villi have a continuous membranous covering. This layer is extremely thin, and CSF passes through the villi at a linearly increasing rate as CSF pressures rise above 68 mm H2O. This passive route is not the only manner in which CSF water and other contents are transported. Tripathi and Tripathi, in serial electron micrographs, found that the mesothelial cells of the arachnoid villus continually form giant cytoplasmic vacuoles that are capable of transcellular bulk transport. Certain substances, such as penicillin and organic acids and bases, are also absorbed by cells of the choroid plexus; the bidirectional action of these cells resembles that of the tubule cells of the kidneys. Some substances have been shown in pathologic specimens to pass between the ependymal cells of the ventricles and to enter subependymal capillaries and venules.
By infusing and withdrawing CSF under controlled circumstances, the resistance to CSF absorption and its rate of replacement can be calculated. The resistance to the passage of CSF into the venous system has been termed R0 and can be expressed in terms similar to the Ohm law (E = IR): the voltage (E) reflects the difference in pressure between the CSF and the venous system (PCSF – PV), which drives CSF into the dural sinuses, and the equivalent of electrical current, termed If, represents the flow rate of CSF. In the steady state, this flow rate is equal to the rate of CSF production (0.3 mL/min). R0, the resistance to absorption, which under normal circumstances is approximately 2.5, rises when there is a blockage in the CSF circulation. The equation for CSF pressure can therefore be expressed as PCSF − PV = If × R0; when rearranged, PCSF = PV + If × R0. Because the product of If × R0 is only 0.8 mm Hg, it can be appreciated that the main contribution to CSF pressure—as measured by spinal puncture—is the venous pressure, PV. Restated, the intracranial pressure and CSF pressure, which are in equilibrium, are derived predominantly from transmitted vascular pressures and not from CSF outflow resistance. However, in pathologic conditions such as bacterial meningitis and subarachnoid hemorrhage, R0 may rise to levels that impede CSF circulation, raise pressure.
In the recumbent position, ICP and, consequently, CSF pressure are normally about 8 mm Hg or 110 mm H2O (1 mm Hg equals 13.7 mm H2O), with an upper limit of normal that is higher in children than in adults according to Huh and colleagues. As the head and trunk are progressively elevated, the weight of the column of CSF is added incrementally to the pressure in the lumbar subarachnoid space, and the intracranial CSF pressure drops correspondingly, so that it is close to zero in the standing position. As described above, the CSF pressure is in equilibrium with the capillary and prevenous vascular pressures, which are influenced mainly by circulatory changes that alter arteriolar tone. Elevations in systemic arterial pressure cause little or no increase of pressure at the capillary level because of cerebrovascular autoregulation, and hence, little increase in CSF pressure. Extremely low blood pressure (BP), in the range of a mean of 40 mm Hg, however, cannot be compensated for and correspondingly, lower the CSF pressure (which is, of course, zero when the heart stops).
In contrast to the limited effect caused by changes in arterial BP, increased venous pressure exerts an almost immediate effect on CSF pressure by increasing the volume of blood in the cerebral veins, venules, and dural sinuses. If the jugular veins are compressed, there is a rise of ICP that is transmitted to the lumbar subarachnoid space (unless there is a spinal subarachnoid block). This was the basis of the now little used Queckenstedt test, mentioned in Chap. 2. In the case of a spinal block, pressure on the abdominal wall, which affects the spinal veins below the point of subarachnoid block, will still increase the lumbar CSF pressure. The Valsalva maneuver, as well as coughing, sneezing, and straining, causes an increased intrathoracic pressure, which is transmitted to the jugular and then to the cerebral and spinal veins and directly to the CSF compartment through the intervertebral foramina. The jugular venous valves prevent unimpeded transmission of pressure to the cranial veins but this threshold can be exceeded if central and jugular venous pressures become greatly elevated. Mediastinal tumors, by obstructing the superior vena cava, have the same effect.
The inhalation or retention of CO2 raises the blood Pco2 and correspondingly decreases the pH of the CSF. By a mechanism that is not fully understood, this acidification of the CSF acts as a potent cerebral vasodilator, causing an increase in cerebral blood flow and blood volume, thus leading to intracranial hypertension. Hyperventilation, which reduces Pco2, has the opposite effect; it increases the pH and the cerebral vascular resistance and thereby decreases CSF pressure; this maneuver of lowering the arterial CO2 content by hyperventilation is used in the treatment of acutely raised ICP.
Disturbances of Cerebrospinal Fluid Pressure, Volume, and Circulation
The intact cranium and vertebral canal, together with the relatively inelastic dura, form a rigid container, such that an increase in the volume of any of its contents—brain, blood, or CSF—will elevate the ICP. Furthermore, an increase in any one of these components must be at the expense of the other two, a relationship that is known as the Monro-Kellie doctrine. Small increments in brain volume do not immediately raise the ICP because of the countervailing buffering effect of displacement of CSF from the cranial cavity into the spinal canal. To a lesser extent, there is deformation of the brain and stretching of the infoldings of the relatively unyielding dura, specifically, the falx cerebri between the hemispheres and the tentorium between the hemispheres and cerebellum. Once these compensating measures have been exhausted, a mass within one dural compartment leads to displacement, or “herniation” of brain tissue from that compartment into an adjacent one. Any further increment in brain volume necessarily reduces the volume of intracranial blood contained in the veins and dural sinuses. Also, the CSF is formed more slowly in circumstances of raised ICP. These accommodative volume-pressure relationships occur concurrently and are subsumed under the term intracranial elastance (the change in ICP for a given change in intracranial volume, or its inverse, compliance). As the volume of brain, blood, or CSF continue to increase, the accommodative mechanisms fail and ICP rises exponentially, as in an idealized elastance curve. The normal curve begins its steep ascent at an ICP of approximately 25 mm Hg. After this point, small increments in intracranial volume result in marked elevations in ICP.
The numerical difference between ICP and mean BP within the cerebral vessels is termed cerebral perfusion pressure (CPP). Besides the aforementioned brain tissue shifts, which are discussed more fully in relation to their clinical signs in Chap. 17, elevation in ICP that approaches the level of mean systemic BP eventually causes a widespread reduction in cerebral blood flow. In its most severe form, this results in global ischemia and produces brain death. Lesser degrees of raised ICP and reduced cerebral perfusion cause correspondingly less severe, but still widespread, cerebral infarction that is similar to what arises after cardiac arrest. In all circumstances, not only the severity but also the duration of reduced cerebral perfusion, are the main determinants of the degree of cerebral damage. These are theoretical models that guide practice but are often found to be imprecise in clinical circumstances.
Lundberg has been credited with recording and analyzing intraventricular pressures over long periods of time in patients with brain tumors. He found ICP to be subject to periodic spontaneous fluctuations, of which he described three types of pressure waves, designated as A, B, and C. Only the A waves have proved to be separable from arterial and respiratory pulsations and are of clinical consequence. They consist of rhythmic wave-like elevations of ICP, up to 50 mm Hg, occurring every 15 to 30 min and lasting about 1 min, or of smaller but more protracted elevations. These plateau waves, as they have come to be known, coincide with an increase in intracranial blood volume, presumably as a result of a temporary failure of cerebrovascular autoregulation. Rosner and Becker have observed that plateau waves are sometimes preceded by a brief period of mild systemic hypotension. In their view, this slight hypotension induces cerebral vasodilatation in order to maintain normal blood flow. Upon recovery of the BP, the response in cerebrovascular tone is delayed, thereby allowing intracranial blood volume to accumulate in the dilated vascular bed, and raising ICP in the form of a plateau wave. In support of this explanation is the observation that a brief period of induced elevation of BP paradoxically restores the normal cerebrovascular tone and leads to an abrupt cessation of a plateau wave.
The high rates of mortality and morbidity of acute cerebral mass lesions is in part related to uncontrolled elevation in ICP. In a normal adult reclining with the head and trunk elevated to 45 degrees, the ICP is in the range of 2 to 5 mm Hg. Levels above 15 mm Hg that are considered abnormal are not in themselves hazardous; in fact, adequate cerebral perfusion can be maintained at an ICP of 40 mm Hg provided BP remains normal. A higher ICP or a lower BP may combine to reduce CPP and cause diffuse ischemic damage.
In clinical practice, there are several mechanisms for elevated ICP:
A cerebral or extracerebral mass such as brain tumor; cerebral infarction with edema; traumatic contusion; parenchymal, subdural, or extradural hematoma; or abscess, all of which tend to be localized and deform the adjacent brain. The brain deformation is greatest locally, being compartmentalized to a varying degree by dural partitions.
Generalized brain swelling, as occurs in ischemic–anoxic states, acute hepatic failure, hypertensive encephalopathy, hypercarbia, and the Reye hepatocerebral syndrome. In these disorders, the increase in pressure can reduce cerebral perfusion, but tissue shifts are minimal because the mass effect is uniformly distributed throughout the cranial contents.
An increase in venous pressure because of cerebral venous sinus thrombosis, heart failure, or obstruction of the superior mediastinal or jugular veins.
Obstruction to the flow and absorption of CSF. If the obstruction is within the ventricles or in the subarachnoid space at the base of the brain, hydrocephalus results. Extensive meningeal disease from any number of causes (infectious, carcinomatous, granulomatous, hemorrhagic) is another mechanism of blockage to CSF flow. If the block is confined to the absorptive sites adjacent to the cerebral convexities and superior sagittal sinus, the ventricles remain normal in size or enlarge only slightly, because the pressure over the convexities approximates the pressure within the lateral ventricles (see further on).
Any process that expands the volume of CSF (meningitis, subarachnoid hemorrhage) or increases CSF production (choroid plexus tumor). In this situation, there may be a pressure gradient between the cerebral and spinal compartments, resulting in hydrocephalus.
The clinical manifestations of increased ICP in children and adults are headache, nausea and vomiting, drowsiness, ocular palsies, and papilledema. Papilledema may result in periodic visual obscurations; if it is protracted, optic atrophy and blindness may follow (see Chap. 13 for further discussion of papilledema and optic nerve atrophy). The practice of monitoring ICP with a pressure device inserted into the cranial cavity has permitted approximate correlations to be made between clinical signs and the levels of ICP. However, the main neurologic signs of a large intracranial mass, pupillary dilatation, abducens palsies, drowsiness, and the Cushing response, as discussed below and in Chaps. 17 and 35, are caused by displacement of brain tissue, and therefore do not bear a strict relationship to ICP.
As a rule, patients with normal BP retain normal mental alertness with ICP of 25 to 40 mm Hg unless there is concurrent shift of brain tissue. Stated another way, coma generally cannot be attributed to elevated ICP alone. Only when ICP exceeds 40 to 50 mm Hg does cerebral blood flow diminish to a degree that results in loss of consciousness. Any further elevations are followed imminently by global ischemia and brain death. From our own observations, the brain shift and herniation that causes the pupil to dilate on the side of a mass lesion generally occurs at an ICP of 28 to 34 mm Hg. (Again, this is not a direct effect of the elevated ICP.) There are instances in which the dissociation between ICP and clinical signs is striking, for example, a mass in the medial temporal lobe that is contiguous to the third nerve will compress the nerve at a time when pressure is little raised, and slowly growing lesions, which raise ICP slowly and allow for compensation cause few clinical signs. Likewise, unilateral or bilateral abducens palsies do not have a uniform relationship to the degree of elevation of ICP. Rather, abducens palsies are most frequent when raised ICP is a result of diffusely distributed brain swelling, hydrocephalus, meningitic processes, or pseudotumor states.
The consequences of increased ICP take on special features in infants and small children, whose cranial sutures have not closed. The head enlarges and the eyes may bulge. Then the clinical problem involves differentiation from other types of enlargement of the head with or without hydrocephalus, such as constitutional macrocrania or an enlarged brain (megalencephaly; or hereditary metabolic diseases such as Krabbe disease, Alexander disease, Tay-Sachs disease, Canavan spongy degeneration of the brain), and from subdural hematoma or hygroma, neonatal ventricular hemorrhage, and various cysts and tumors.
There is evidence, mainly from retrospective cohorts of patients with traumatic brain injury, that the outcome in patients with intracranial mass lesions is better if the ICP is maintained at levels well below those that compromise cerebral perfusion. An ICP below 15 or 20 mm Hg is often given as a therapeutic target, as the elastance curve becomes steep beyond this level and small increments in cerebral volume may cause large elevations in pressure. However, guiding treatment by the direct measurement of ICP has not yielded uniformly beneficial results, and—after several decades of popularity—the advantage of such devices over clinical and imaging signs of increasing mass effect, is not certain. Nonetheless, in a patient who cannot be examined because of induced paralysis or sedation, or who will be subjected to a procedure that risks further elevating ICP, monitoring seems sensible.
The problem in demonstrating an advantage to monitoring may partly be a matter of the level of ICP at which treatment is instituted and the proper selection of patients for treatment. Contributing to the decision to institute monitoring are the prospects of ameliorating the underlying lesion, the patient’s age, and associated medical disease(s). Our practice has been to measure ICP with an indwelling fiberoptic monitor or intraventricular catheter in otherwise salvageable patients who are stuporous or comatose, and in whom a traumatic or other intracranial mass has caused either a shift of intracranial structures or severe generalized brain swelling. Published guidelines suggest that monitoring should be undertaken in patients with severe traumatic brain injury if they are over 40 years of age, and have a Glasgow Coma Score below 9 (see Chap 35). The monitor is generally placed on the same side as a mass lesion. Whether recent studies, such as the one by Chesnut and colleagues comparing monitoring to clinical and imaging in directing therapy, negate these guidelines is also uncertain.
The emergency management of raised ICP is considered in greater detail in Chaps. 34 and 35 where the subject is discussed in relation to stroke and cerebral trauma. A comprehensive review can be found in the monograph on intensive care (Ropper) listed in the references.
This is a condition of ventricular enlargement as a result of an obstruction to the normal flow of CSF. The sites of obstruction may be at the third ventricle, aqueduct of Sylvius, at the medullary foramina (Luschka and Magendie), or in the basal or convexity subarachnoid spaces. Because of the obstruction, CSF accumulates within the ventricles under increasing pressure, enlarging the ventricles, and expanding the hemispheres. As stated earlier, in the infant or young child, the head increases in size because the expanding cerebral hemispheres separate the sutures of the cranial bones.
Regarding terminology, the term hydrocephalus (literally, “water brain”) is frequently but incorrectly applied to any enlargement of the ventricles, even if consequent to cerebral atrophy, i.e., hydrocephalus ex vacuo, or to ventricular enlargement because of failure of development of the brain, a state known as colpocephaly. Reference to these conditions as hydrocephalic is so common that it is unlikely to change; the use of “tension hydrocephalus,” although not widely adopted, separates enlargement caused by pressure from passive enlargement of the ventricles.
In 1914, Dandy and Blackfan introduced the also somewhat inaccurate but now well-established terms communicating and noncommunicating (obstructive) hydrocephalus. The concept of communicating hydrocephalus was based on the observations that dye injected into a lateral ventricle would diffuse readily downward into the lumbar subarachnoid space and that air injected into the lumbar subarachnoid space would pass into the ventricular system; in other words, the ventricles were in communication with the spinal subarachnoid space. If the lumbar spinal fluid remained colorless after the injection of dye, the hydrocephalus was assumed to be obstructive, or noncommunicating. In actuality, the distinction between these two types is not fundamental, because all forms of true hydrocephalus are obstructive at some level, and the obstruction is virtually never complete.
There are several sites of predilection of obstruction to the flow of CSF. One foramen of Monro may be blocked by a tumor or by horizontal displacement of central brain structures from a large cerebral mass. This obstruction may cause expansion of the lateral ventricle or a portion of it. Tumors within the third ventricle (e.g., colloid cyst) block the egress of CSF from both foramina of Monro, leading to dilatation of both lateral ventricles. The aqueduct of Sylvius, narrow to begin with, may be occluded by a number of developmental or acquired lesions (genetically determined atresia or forking, perinatally acquired gliosis, ependymitis, hemorrhage, tumor), and lead to dilatation of the third and both lateral ventricles. If the obstruction is in the fourth ventricle, the dilatation includes the aqueduct. Other sites of obstruction of the CSF in the ventricular pathways are at the foramina of Luschka and Magendie (e.g., congenital failure of opening of the foramina, Dandy-Walker syndrome). More common is a blockage to flow of CSF in the subarachnoid space around the brainstem caused by inflammatory or fibrosing meningitis. This form of obstruction results in enlargement of the entire ventricular system, including the fourth ventricle. A useful adage, attributed to Ayer, is that the ventricle closest to the obstruction enlarges the most; meaning, for example, that occlusion of the basal CSF pathways causes a disproportionate enlargement of the fourth ventricle, and a mass within the fourth ventricle leads to a greater dilatation of the third than of the lateral ventricles.
Another potential site of obstruction is in the subarachnoid spaces over the cerebral convexities. A matter of considerable practical as well as theoretical interest is whether a meningeal obstruction at the site of the arachnoidal villi, or a blockage of the venous sinuses into which the CSF is absorbed, can result in hydrocephalus. Russell, in her extensive neuropathologic material and review of the world’s literature, could not find a well-documented example of either of these suggested etiologies, and the same is true of the pathologic material collected by our colleague R.D. Adams. Moreover, experiments in animals in which all the draining veins had been occluded, tension hydrocephalus with enlarging lateral ventricles was produced in only a few cases. Yet Gilles and Davidson have stated that hydrocephalus in children may be the result of a congenital absence, or deficient number of arachnoidal villi, and Rosman and Shands have reported an instance that they attributed to increased intracranial venous pressure. Our hesitation in accepting such examples stems from the difficulty that the pathologist has in judging the patency of the basilar subarachnoid space. This is much more reliably visualized by radiologic than by neuropathologic means. Theoretically, as mentioned earlier, if the obstruction is high, near (or in) the superior sagittal sinus, the CSF should accumulate under pressure outside as well as inside the brain, and the ventricles should not enlarge at all or only slightly, and only after a prolonged period.
The rarely encountered radiologic picture of enlarged subarachnoid spaces over and between the cerebral hemispheres, coupled with modest enlargement of the lateral ventricles has been referred to as external hydrocephalus. Although such a condition does exist, many of the cases so designated have proved to be examples of subdural hygromas or arachnoid cysts. The condition is now seen somewhat more regularly after surgical hemicraniectomy if the bone is not replaced.
Processes such as subarachnoid hemorrhage, or cerebral hemorrhage or brain abscess that rupture into the ventricles and rapidly expand the volume of CSF produce the most dramatic forms of acute hydrocephalus. The obstruction of the CSF pathways may also be found within the ventricular system or at the basal meninges. The corresponding clinical syndrome is described below.
An increase in the rate of formation or decrease in the rate of absorption would be expected to cause an accumulation of CSF and increased ICP. The only reported examples of overproduction of CSF are papillomas of the choroid plexus, but even in this circumstance, there is usually an associated ventricular obstruction, either of the third or fourth ventricle or of one lateral ventricle. Characteristically in these cases, there is both a generalized dilatation of the ventricular system and basal cisterns (possibly because of increased CSF volume), and an asymmetrical enlargement of the lateral ventricles caused by obstruction of one foramen of Monro.
This process gives rise to various syndromes depending on the age of the patient and the rapidity of evolution. One type occurs very early in life, before fusion of the cranial sutures and causes enlargement of the head. If the cranial sutures have fused, the head remains normal in size and the ventricular enlargement may remain asymptomatic or cause gait, urinary and cognitive difficulties. A special form of the latter is arrested or compensated hydrocephalus of late adult life that is one of the causes of normal-pressure hydrocephalus that is addressed in Chap. 7 and extensively below.
The cranial bones fuse by the end of the third year; for the head to enlarge, the tension hydrocephalus must develop before this time. It may begin in utero, but usually happens in the first few months of life. Even up to 5 years of age (and very rarely beyond this time), a marked increase of ICP, particularly if it evolves rapidly, may separate the newly formed sutures (diastasis). Tension hydrocephalus, even of mild degree, also molds the shape of the skull in early life, and in radiographs, the inner table is unevenly thinned, an appearance referred to as “beaten silver”, or as convolutional or digital markings. The frontal skull regions are unusually prominent (bossed) and the skull tends to be brachiocephalic, except in the Dandy-Walker syndrome where, because of bossing of the occiput from enlargement of the posterior fossa, the head is dolichocephalic. With marked enlargement of the skull, the face looks relatively small and pinched, and the skin over the cranial bones is tight and thin, revealing prominent distended veins.
The usual causes of this disorder are (1) intraventricular matrix hemorrhages in premature infants, (2) fetal and neonatal infections, (3) type II Chiari malformation, (4) aqueductal atresia and stenosis, and (5) the Dandy-Walker syndrome.
In congenital hydrocephalus, the head usually enlarges rapidly and soon surpasses the ninety-seventh percentile. The anterior and posterior fontanels are tense even when the patient is in the upright position. The infant is fretful, feeds poorly, and may vomit frequently. With continued enlargement of the brain, torpor sets in and the infant appears languid, uninterested in his surroundings, and unable to sustain activity. Later it is noticed that the upper eyelids are retracted, and the eyes tend to turn down; there is paralysis of upward gaze, and the sclerae above the irises are visible. This is the “setting-sun sign” that has been incorrectly attributed to downward pressure of the frontal lobes on the roofs of the orbits. The fact that it disappears on shunting the lateral and third ventricles indicates that it is caused by hydrocephalic pressure on the mesencephalic tegmentum. Gradually, if left untreated, the infant adopts a posture of flexed arms and flexed or extended legs. Signs of corticospinal tract damage are usually elicitable. Movements are feeble, and sometimes the arms are tremulous. There is no papilledema, but later the optic discs become pale and vision is reduced.
If the hydrocephalus becomes arrested, the infant or child is usually developmentally delayed in motor function but often surprisingly verbal. The head may be so large that the child cannot hold it up and must remain in bed. If the head is only moderately enlarged, the child may be able to sit but not stand, or stand but not walk. If ambulatory, the child is clumsy. Acute exacerbations of hydrocephalus or a febrile illness may cause vomiting, stupor, or coma.
The special features of congenital hydrocephalus associated with the Chiari malformation, aqueductal atresia and stenosis, and the Dandy-Walker syndrome are discussed in Chap. 38. Also mentioned here is a rare condition termed “bobble head” syndrome that is caused by a suprachiasmatic arachnoid cyst or by third ventricular enlargement, as discussed in Chap. 31. These lesions cause the child’s head to move forward and backward or side-to-side constantly or intermittently at about 2 to 3 Hz. There is no direct connection to another rhythmic head movement, spasmus nutans (listed in some books as “mutans”), or to seesaw nystagmus described in Chap. 14, but the location of the causative lesions, in and adjacent to the third ventricle, is similar in all of them.
Here, the ventricular enlargement becomes evident only after the cranial sutures have closed (Fig. 30-2). The causes of obstruction to the flow of CSF are diverse, and although some are clearly congenital, symptoms may be delayed as late as adolescence, or early adult life, or even later. In some instances, the condition gives rise to normal-pressure hydrocephalus, as discussed below and in Chap. 7. The clinical features of occult hydrocephalus and the course of the illness are quite variable. We have seen a few cases in adults in whom the gait disturbance from congenital aqueductal stenosis appeared abruptly enough to give the impression of a cerebellar or frontal stroke. For unexplained reasons, the symptoms of previously occult hydrocephalus may also appear abruptly after minor cranial trauma. A suck reflex and grasp reflexes of the hands and feet are variably present; plantar reflexes are sometimes extensor. Last, there may be urinary urgency leading to sphincteric incontinence, often without the patient’s awareness.
Chapter 31 discusses occult hydrocephalus caused by intracranial tumor growth.
Surprisingly, little has been written about this syndrome despite its frequency in clinical practice. It is seen most often following subarachnoid hemorrhage from a ruptured aneurysm, less often with bleeding from an arteriovenous malformation or from deep intracerebral hemorrhage that dissects into the ventricles; it may also occur as the result of obstruction of the CSF pathways in the fourth ventricle by a tumor or cerebellar–brainstem hemorrhage, or within the basal cisterns by neoplastic infiltration of the meninges, although this last process tends to evolve more subacutely. The patient complains of a headache of varying severity and often of visual obscuration, may vomit, and then becomes drowsy or stuporous over a period of minutes or hours. Bilateral Babinski signs are the rule, and in the advanced stages, which are associated with coma, there is increased tone in the lower limbs and extensor posturing. Early in the process, the pupils are normal in size and the eyes may rove horizontally; as the ventricles continue to enlarge, the pupils become miotic, the eyes then cease roving and assume an aligned position, or there may be bilateral abducens palsies and limitation of upward gaze. The speed with which hydrocephalus develops determines whether there is accompanying papilledema.
If this condition is left untreated, the pupils eventually dilate symmetrically, the eyes no longer respond to oculocephalic maneuvers, and the limbs become flaccid. Or, there is an unanticipated cardiac or respiratory arrest, even at an early stage of evolution of the hydrocephalus; this complication is seen particularly in children with masses, particularly at the foramen magnum, and may be presaged by brain compression at the level of the perimesencephalic cisterns, detectable by imaging studies.
Treatment is by drainage of CSF, usually effected by a ventricular catheter or, if there is undoubted communication between all the CSF compartments, by lumbar puncture. The latter may pose some risk if spinal fluid is withdrawn rapidly or there is a sizeable leak of fluid through the spinal dura at the site of the puncture, thereby creating a pressure gradient between the cerebral and spinal regions.
Ventricular expansion tends to be maximal in the frontal horns, explaining the hydrocephalic impairment of frontal lobe functions and of basal ganglionic–frontal motor and gait activity in most forms of hydrocephalus. The central white matter yields to pressure, while the cortical gray matter, thalami, basal ganglia, and brainstem structures remain relatively unaffected. There is an increase in the content of interstitial fluid in the tissue adjacent to the lateral ventricles (transependymal flow), readily detected by MRI (see Fig. 30-2). Myelinated fibers and axons are injured, but not to the extent that one might expect from the degree of compression; minor degrees of astrocytic gliosis and loss of oligodendrocytes in the affected tissue are present to a decreasing extent away from the ventricles and represent a chronic hydrocephalic atrophy of the brain. The ventricles are characteristically denuded of ependyma, and the choroid plexuses are flattened and fibrotic. The lumens of cerebral capillaries in biopsy preparations are said to be narrowed—a finding that is difficult to evaluate.
Normal-Pressure Hydrocephalus
In meningeal and ependymal diseases, hydrocephalus may develop and reach a stable state. It is then said to be “compensated” in the sense that formation of CSF equilibrates with absorption. Once equilibrium is attained, the ICP gradually falls, though it still maintains a gradient from ventricle to basal cistern to cerebral subarachnoid space. A stage is reached where the CSF pressure reaches a high normal level of 150 to 200 mm H2O, but the patient nonetheless eventually manifests the cerebral effects of the hydrocephalic state. The name given to this condition by Adams, Fisher, and Hakim was normal-pressure hydrocephalus (NPH).
A triad of clinical findings is characteristic of NPH: a slowly progressive gait disorder is usually the earliest feature, followed by impairment of mental function and later, sphincteric incontinence. The reader should be alerted to the fact that the fully developed triad is not usually present in the early stages of the process. Grasp reflexes in the feet and falling attacks may also occur, but there are no Babinski signs. Headaches are infrequently a complaint, and there is no papilledema.
The gait disturbance that accompanies NPH may be of several different types, as discussed in Chap. 7. These are difficult to classify and only vaguely simulate the patterns observed in Parkinson disease or cerebellar ataxia, but certain features predominate. Most often, there is unsteadiness and impairment of balance and shortening of the step length, with the greatest difficulty being encountered on stairs and curbs (Fisher
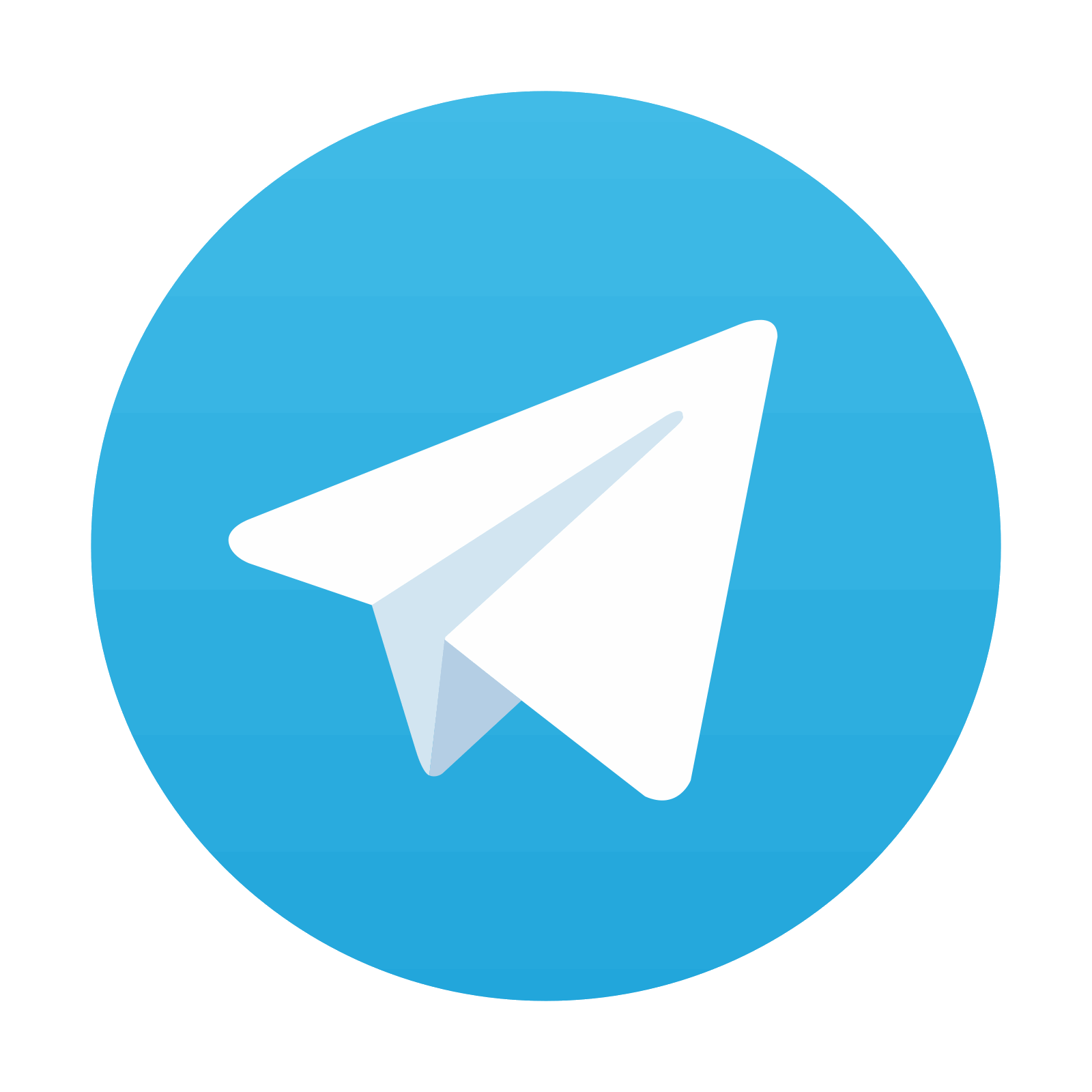
Stay updated, free articles. Join our Telegram channel
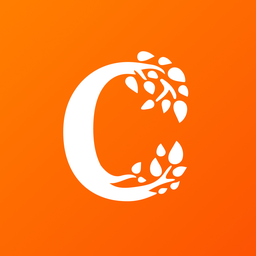
Full access? Get Clinical Tree
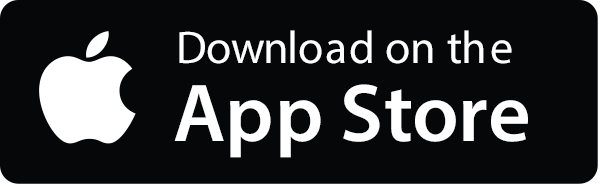
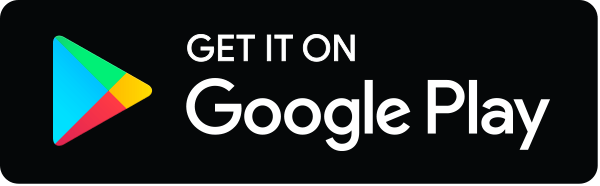
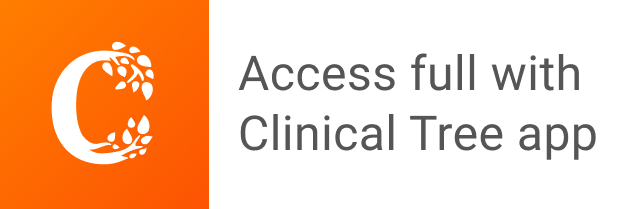