Chapter 32 Circadian Rhythms in Mammals
Formal Properties and Environmental Influences
The Nature of Circadian Rhythms
A salient characteristic of sleep in humans is its daily rhythmicity. A similar 24-hour rhythmicity characterizes most aspects of the behavior, physiology, and biochemistry of living organisms. Historically, daily rhythms were interpreted as innate or acquired responses to environmental stimuli, such as light, temperature, and humidity, that vary markedly with time of day. However, as early as 1729, it was reported that the daily rhythm of leaf movements of the heliotrope Mimosa does not depend on continued exposure to the day–night cycle; that is, the rhythm persisted (free-ran) in constant dark (DD). Many studies have since confirmed that daily rhythms in a great variety of species, including humans, free-run, often indefinitely and with impressive precision, despite housing in controlled environments that lack variations in lighting, temperature, food access, or other stimuli (Fig. 32-1).1
Persistence of daily rhythms for many cycles in the absence of environmental time cues suggests that organisms possess one or more endogenous clocklike timekeeping mechanisms. If these mechanisms were biochemical processes within organisms, they might reasonably be expected to show dependence of rate (frequency, the reciprocal of which is cycle duration or period, represented by the Greek letter τ) on tissue temperature, because chemical processes are almost universally temperature-dependent. Yet, for these mechanisms to time behavior and physiology accurately, they must be capable of cycling at a constant rate, despite variations in tissue temperature caused by changes in metabolism (for homeothermic species) or environmental conditions (for heterothermic species). The evidence accumulated in the 1950s that daily rhythms in most organisms show an impressive stability of period across a range of temperatures fueled a debate over whether daily rhythms were truly endogenously generated or whether they reflected a response of the organism to some unknown, periodic environmental “factor X” associated with Earth’s rotation on its axis (e.g., electromagnetic fields2).
Although the mechanism by which daily rhythms maintain a stable period at different temperatures remains to be fully elucidated, the physiologic plausibility of such a mechanism is no longer in doubt.3 The evidence is now overwhelming that daily rhythms are generated by endogenously rhythmic internal clocks. The most compelling behavioral evidence in support of the endogenous clock concept is the observation that daily rhythms in constant conditions typically express stable τs that deviate from the 24-hour periodicity of the solar day and thus would rapidly slide out of phase with any geophysical correlate of planetary rotation. This circadian (Latin, “approximately a day”) periodicity exhibits individual differences around a species-typical mean, usually within the range of 23 to 25 hours. Consequently, individual animals housed separately under identical environmental conditions gradually drift out of synchrony with local time and with each other. Moreover, shorter (than 24 hours) or longer (than 24 hours) circadian τs (reflecting faster or slower running circadian clocks, respectively) can be promoted by selective breeding or induced by gene mutations (see Chapter 12). A variety of drugs, hormones, environmental manipulations, and processes associated with aging can also modulate clock periodicity (discussed further later). All of these observations are consistent with the hypothesis that there is a genetically specified internal circadian clock that drives daily rhythms in behavior and physiology. Indeed, circadian rhythms are known to persist in constant conditions on space shuttles in orbit, completely removed from any hypothetical terrestrial factor X.4
Over the past few decades, circadian clocks have been localized in the brains and other tissues of several species, and genes and proteins that comprise the gears of these clocks have now been identified in cyanobacteria, a fungus, insects, fish, and mammals (for references, see Chapter 12). Thus, the focus of research in this area is no longer on whether circadian rhythms are generated by internal clocks but, rather, what the components and processes of these clocks are, how they control behavior and physiology, how they are synchronized to the environment, and what impact they have on a variety of medical conditions. In addition, a major current focus is on understanding how our internal clocks might be manipulated to treat problems related to circadian timing, such as the disturbances of sleep, arousal, and other physiologic processes associated with shift work, rapid transmeridian jet travel, blindness, and other conditions. An understanding of the functional properties of circadian clocks is essential to set the framework for the cellular and molecular analyses that address these important questions. This chapter reviews the basic functional properties of circadian clocks, and subsequent chapters review the neurobiological and molecular genetic analyses of these clocks.
Parameters and Measurement of Circadian Rhythms
Overt circadian rhythms have phase and amplitude dimensions (Fig. 32-2). Phase (conventionally represented by the Greek letter φ) refers to any point within a cycle. An observable phase (e.g., daily wakeup time) can be used as the hand of a clock to track its motion. The elapsed time between successive occurrences of a particular phase represents τ. The φ and τ of an observed circadian rhythm reflect parameters of the underlying circadian clock, namely, its current position and its rate of cycling, respectively. Amplitude refers to the range of values that an overt circadian rhythm can assume through the course of its cycle. One measure of amplitude is the difference between the peak value and the mean value of a purely sinusoidal rhythm (the acrophase of a fitted cosine wave; see Fig. 32-2). However, the amplitude of an overt rhythm cannot be taken to represent a parameter of the underlying clock, because many factors downstream from the clock can affect the level and range of particular observable behavioral or physiologic variables.
The experimental analysis of circadian rhythms has relied heavily on a few organisms that exhibit precise and easily measured circadian rhythms (e.g., wheel running, body temperature, plasma melatonin) or that provide advanced molecular genetic resources. Behavioral and physiologic data are typically acquired by sensors whose outputs are monitored continuously by computers. Data stored in digital format are amenable to time-series analyses (e.g., linear regression, spectral analysis) using a variety of established techniques to measure φ and τ and to curve-fitting procedures (e.g., cosinor analysis, see Fig. 32-2) to measure φ and amplitude.5–7 Despite the broad range of species studied and variables monitored, the formal properties of circadian rhythms are remarkably general, a feature consistent with an ancient origin for circadian clocks. In this chapter, we refer primarily to the literature from studies of mammalian circadian rhythms.
Adaptive Significance of Circadian Rhythms
Circadian clocks are thought to have several important functions that enhance reproductive fitness in natural environments. A primary function is to promote consolidated periods of activity (active engagement with the environment) and rest (withdrawal from environmental engagement), and to restrict these to appropriate phases of the solar day, resulting in nocturnal (night-active), diurnal (day-active) or crepuscular (dawn- and dusk-active) chronotypes that are consistent with the sensory and other adaptations of a given species. A fundamental advantage of using an internal clock to regulate rest–activity cycles is that physiologic changes can be mobilized in anticipation of the transitions between day and night that herald the dramatic ecologic differences between these daily phases. This allows an anticipatory, rather than reactive, regulation of physiology, thus ensuring that the organism is fully prepared for activity or rest at the correct time. Humans and other diurnal species thus exhibit a gradual increase in body temperature, plasma cortisol, and sympathetic autonomic tone beginning several hours before habitual wake onset, thereby facilitating a rapid switch from sleep to alert waking8 (see Chapter 35). Similar preparatory processes occur in reverse to facilitate sleep onset (e.g., body temperature declines, melatonin secretion begins).
In at least some species, the circadian clock has been exploited not only as a device for driving daily rhythms (i.e., a pacemaker function) but also as a true clock that can be continuously consulted to provide a sense of time. Evidence for this function is available from studies showing that some animals can discriminate time of day without external cueing and thereby learn to associate the time and place of food availability.9,10 Some species use this time sense to navigate using celestial cues as a compass, which requires continuous adjustment of the angle of movement relative to the sun’s apparent position in the sky.11 The circadian clock is also used by many species to measure day length for the purposes of regulating seasonal reproduction, hibernation, and other functions for which anticipation is critical.12
Environmental Influences
Photic Entrainment
Effects of Light on Circadian Phase
To achieve the adaptive functions of pacemaker and continuously consulted clock, it is imperative that the circadian clock maintain an appropriate phase relation to local environmental time. This challenge is complicated by seasonal variations in day length; outside the equatorial zones, the hours of dawn and dusk shift systematically each day as Earth rotates on its axis and tilts slowly toward and away from the sun. The circadian clock therefore has two tasks: it must generate a cycle that approximates the period of the day–night cycle, and it must track a particular phase of the day–night cycle, so that diurnal animals become active near dawn and nocturnal animals become active near dusk.13 The process by which this optimal synchronization occurs is called entrainment, defined as phase and period control of one oscillating process by another. In the case of the daily sleep–wake cycle and other circadian rhythms, entrainment to local environmental time is mediated by the actions of periodic environmental stimuli (zeitgebers, German for “time-givers”) on the circadian clock.
As might be expected, light is a virtually universal zeitgeber for the circadian rhythms displayed by most organisms, from cyanobacteria to mammals. The mechanism by which light entrains circadian rhythms has been investigated by assessing the effects of discrete applications of light on circadian rhythms free-running in DD.14 Brief light pulses cause phase shifts of free-running rhythms whose magnitude and direction depend on when within the circadian cycle the light pulse is presented (Fig. 32-3A and B). This phase dependence of light effects is illustrated graphically by plotting the size and direction of the phase shift against the circadian phase of light presentation, producing a phase-response curve (PRC) for light (see Fig. 32-3C).
The shape of the PRC for light pulses suggests that entrainment can be accomplished by a phase-delaying action of light at dusk and a phase-advancing action at dawn, producing a net daily shift equal to the difference between τ of the circadian pacemaker and the period (T) of the light–dark cycle (24 hours in natural environments). Consistent with this hypothesis, known as the discrete (or nonparametric) model of entrainment,13 organisms can be entrained to photoperiods consisting of only two brief light pulses in each 24-hour cycle, simulating dawn and dusk (see Fig. 32-3F). Stable entrainment under such skeleton photoperiods has been documented in several species and appears to be similar in most respects to entrainment under a full photoperiod. In fact, studies of nocturnal rodents in seminatural environments indicate that self-selected brief exposures to light at dawn or dusk (or both) might account fully for entrainment in these species.15
The τ of a circadian pacemaker and its circadian rhythm of sensitivity to light (summarized quantitatively in the PRC) determine several important properties of entrainment. One property is the limited range of entrainment. The circadian clock can entrain to light–dark cycles only if there is a relatively close match between τ and T of the light–dark cycle. For stable entrainment to occur, the difference between τ and T generally cannot exceed the maximum daily phase shift that can be induced by exposure to available light. Thus, entrainment of humans to the solar day on Mars (24.6 hours) is feasible (assuming a τ of 24.2 hours, this requires a 24 min delay each day), whereas stable entrainment to the 18-hour duty cycle on an American submarine crew should be virtually impossible (a 6-hour advance being required each circadian cycle16).
A second property of entrainment that is determined by τ, T, and PRC shape is the phase relation between rhythm and zeitgeber during stable entrainment (see Fig. 32-2C). The phase relation, or phase difference (abbreviated Ψ), is the difference in minutes (or radial degrees, if expressed as a phase angle) between a definable circadian rhythm phase (e.g., wake onset) and a definable zeitgeber phase (e.g., sunrise, in which case the phase relation can be abbreviated ΨLD). Stable entrainment will occur at whatever ΨLD results in the photic stimulation that generates a net phase shift that precisely compensates for the difference between τ and T. If τ is less than 24 hours, then a net phase delay is required each circadian cycle. A larger net delay will occur when evening light stimulates more of the phase-delay portion of the PRC and when morning light stimulates less of the phase-advance portion. This occurs if the PRC (i.e., the circadian clock) is shifted slightly to the left (i.e., earlier) relative to environmental time. Thus, a bird with a short circadian τ assumes a more positive ΨLD, resulting in an earlier onset of activity at dawn. By contrast, if τ longer than 24 hours, a net daily advance is required, stable entrainment will occur at a more negative ΨLD, and wakeup time will occur later relative to dawn. The shape of the PRC of course also plays a role. The case can be made that ΨLD is the ultimate target of natural selection for the value of τ and the shape of the PRC, a premise summarized indirectly in the aphorism that the early bird gets the worm.
Individual differences in τ might also account for the early-bird and night-owl phenotypes that we recognize among humans. In fact, an extreme form of the early-bird phenotype (advanced sleep-phase syndrome) has been traced to a molecular change that affects the periodicity of the human circadian clock,17 and the strongly delayed phenotype of teenagers has been attributed to lengthening of τ during puberty.18 Differences in ΨLD can also occur as a result of small differences in PRC shape or in the strength of the zeitgeber (e.g., the duration, intensity, or wavelength of light exposure each day).
A third important property of entrainment determined by τ and the PRC is the response of circadian rhythms to acute displacements of the light–dark cycle. If the light–dark cycle is advanced by 6 to 9 hours, simulating a rapid trip east from North America to Europe, the circadian clock re-entrains gradually, by small phase shifts (transient cycles) over several days. The size and direction of these shifts is determined by τ; the intensity, wavelength, and precise timing of light; and the shape of the PRC (specifically, the area under the delay and advance portions and the phase at which resetting crosses over from delays to advances). Syrian hamsters have a species-typical τ very close to 24 hours and a relatively large advance-to-delay ratio of their PRC (see Fig. 32-3C). Not surprisingly, they re-entrain to an advance of the light–dark cycle by daily phase advances of about 1 hour (see Fig. 32-3D) and to a delay of light–dark cycle by a series of about 1-hour daily delays. By contrast, Norway rats typically express a τ longer than 24 hours (e.g., see Fig. 32-1B) and re-entrain to an 8-hour light–dark advance by phase delaying 16 hours. The average endogenous τ in humans is slightly longer than 24 hours, which likely contributes in most people to more rapid re-entrainment after flying west (requiring a delay) than east (requiring an advance), and explains why humans might phase delay for many cycles to achieve a large phase advance.19
Effects of Light on Circadian Period
Daily light-induced phase shifts may be critical for adjusting circadian rhythms to large displacements of the light–dark cycle, as, for example, following shift-work rotations or transmeridian jet travel or migration, but quantitative models suggest that phase resetting alone might not be sufficient to explain the precision of observed circadian rhythms during steady-state entrainment.20 Stable, high-precision entrainment might also require modulation of intrinsic pacemaker τ. Plasticity of pacemaker τ has been demonstrated in both light pulse and T-cycle entrainment studies. Light pulses early in the subjective night induce phase-delay shifts and tend to lengthen τ in DD, whereas pulses late in the subjective night induce phase-advance shifts and tend to shorten τ.20 In natural environments, light exposure early in the subjective night would occur when τ is less than 24 hours, and lengthening of τ in response to light would tend to move τ closer to 24 hours. Similarly, light exposure late in the subjective night would occur when τ is longer than 24 hours, and shortening of τ would move it closer to 24 hours. Evidence that such τ matching occurs is provided by T-cycle experiments, which show that τ in DD tends to be longer following entrainment to T longer than 24 hours and tends to be shorter following entrainment to T shorter than 24 hours.21,22 These after-effects on τ can persist for many weeks, and they are likely the basis for the observation that the upper and lower limits of entrainment to T cycles can be extended if the T cycles are gradually lengthened or shortened, respectively.
Experimental analysis of entrainment mechanisms has relied heavily on a few nocturnal rodent species that have remarkably precise wheel-running rhythms, permitting easy measurement of even small changes in phase. Although relatively little work has been done on diurnal mammals, the available light-pulse PRCs and τ-response curves appear to be qualitatively similar to those for nocturnal rodents.20,23,24 Thus, the mechanism of discrete entrainment by daily phase and τ adjustments induced by light exposure at dawn and dusk appears to be available to diurnal species, including humans.25
The photic environments of diurnal and nocturnal animals differ to the extent that diurnal animals are exposed to light throughout the daytime. Whether this additional midday light contributes to entrainment is uncertain. When light is on continuously (LL), simulating the high arctic summer, τ varies systematically with light intensity.26 In nocturnal rodents, LL slows the circadian clock (i.e., τ is lengthened), shortens the daily active phase, and reduces total daily activity (see Fig. 32-5D). In diurnal animals, LL generally has the opposite effects, although there are more exceptions across species (and diurnal primates, in particular, tend not to conform). These empirical generalizations are collectively known as the circadian rule26 and suggest that light could facilitate entrainment by continuous modulation of τ. This forms the basis for a continuous or parametric model of entrainment.20,26
Field studies of a semifossorial diurnal rodent, the European ground squirrel, reveal that they emerge from light-tight burrows several hours after sunrise and return to the burrow several hours before sunset.27 The light–dark cycle is therefore self-selected, which should result in a systematic drift toward dawn or dusk, consistent with an endogenous non–24-hour τ. Instead, they exhibit a precise 24-hour activity rhythm that reflects time of year, with the active phase expanding and contracting with day length across the year, always avoiding dawn and dusk. If the ground squirrel’s rhythm is truly entrained, then its circadian pacemaker must be responsive to light during the day. Although the middle of the day is considered a dead zone, based on studies of brief light pulses in nocturnal species, this feature may be absent from the PRC of some diurnal species, as has been proposed for humans.28
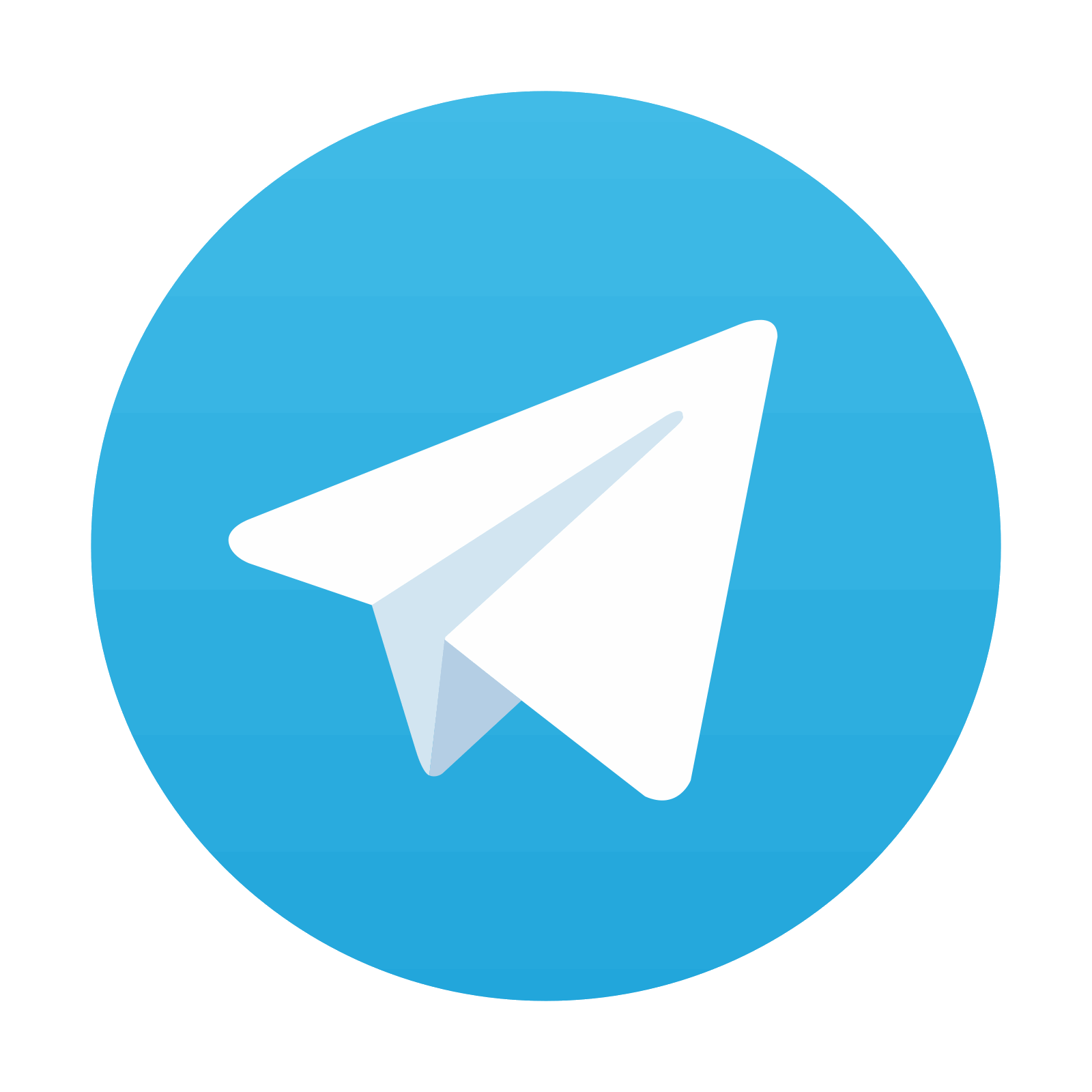
Stay updated, free articles. Join our Telegram channel
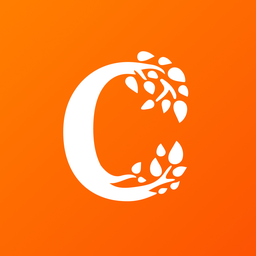
Full access? Get Clinical Tree
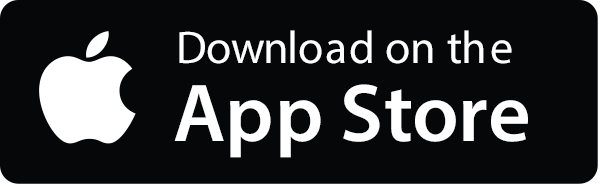
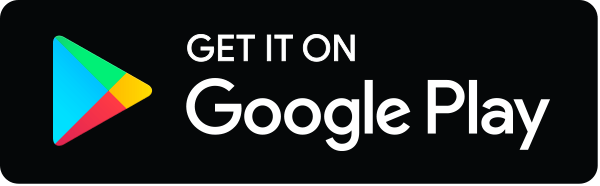