Introduction
Frans Jöbsis first described the use of in vivo tissue near infrared spectroscopy (NIRS) in the human brain in 1977. The technique is based on the concept that light of wavelengths 680 to 1000 nm is able to penetrate human tissue and is absorbed by the chromophores oxyhemoglobin (HbO 2 ) and deoxyhemoglobin (HHb) and the cytochrome oxidase. Changes in the detected light levels therefore can represent changes in concentrations of these chromophores.
The noninvasive nature of the technique, which uses high-intensity light emitted from sources placed on the surface of the scalp, led to its first clinical application in monitoring the cerebral oxygenation status of premature infants. Initially, near infrared spectroscopy NIRS was not quantified and only provided a trend of increased or decreased oxygenation. The early machines were large and cumbersome, and the detected signals showed considerable drift and were prone to movement artifact.
The clinical use of NIRS for monitoring the brain is well established in neonates, in whom transillumination is possible because of the thin skull and small dimensions. However, the clinical application of NIRS to monitor the adult brain has been hampered because it must be applied in reflectance mode. This has resulted in concerns about quantification, the volume and type of tissue being illuminated, and most significantly the issue of signal contamination by the extracranial tissue layers. A number of algorithms have been applied to try to overcome these issues, and techniques such as time-resolved, phase-resolved, and spatially resolved spectroscopy have been developed.
The use of NIRS to evaluate cerebral hemodynamic changes has been reported in a variety of medical and neurosurgical conditions, including those associated with disturbed cerebral circulation. The authors’ experience has shown that in patients with head injury, changes in oxyhemoglobin correlate well with changes in jugular venous oxygen saturation (SjvO 2 ), transcranial Doppler (TCD), and laser Doppler. The authors also have seen that NIRS can provide a more sensitive indicator of desaturation events than SjvO 2 , and a threshold for cerebral ischemia has been defined.
NIRS is an evolving technology, and there has been renewed interest in NIRS as an easy-to-use, noninvasive technique to measure tissue oxygenation in the adult brain. In addition, NIRS use has expanded to examine oxygenation in other tissues and to estimate the adequacy of systemic circulation ; this can be particularly useful in resuscitation from shock or sepsis. Technologic advances have led to the development of compact, portable instruments that detect changes in optical attenuation of several wavelengths of light, with the potential to derive a tissue oxygen saturation from spontaneous HHb and HbO 2 signal changes. NIRS shows promise as a clinical tool in the context of bedside cerebral blood flow measurements, and to image the brain.
This chapter looks at the clinical application of NIRS but stresses the importance of both the assumptions on which NIRS is based, and the limitations of the technology, to interpret the results correctly. It describes the authors’ use of this technique and how it is integrated with other monitoring modalities.
Basic Principles
The theory behind NIRS is described in detail elsewhere, but the basic principle is based on the fact that light in the near infrared range (680-1000 nm) can pass through skin, bone, and other tissues with relative ease. The near infrared light is delivered via fiber-optic bundles, or optodes, placed either on opposite sides of the head, or close together (3.5-6 cm distance) on the same side ( Fig. 33.1 ). Light penetrates the scalp and brain tissue and is subjected to scatter and absorption within the various tissues. A fraction of the photons is captured by the second optode and conveyed to a measuring device.

The concentration of some light-absorbing compounds such as melanin, bilirubin, and water remains virtually constant over time. However, the concentrations of others, such as HbO 2 , HHb, and cytochrome oxidase, vary according to tissue oxygenation and metabolism. Cytochrome oxidase, the terminal enzyme of the respiratory chain, also contributes to the near infrared spectrum of cortical tissue. To calculate the concentration of the chromophobes requires knowledge of the absorption spectra for HbO 2 and HHb, and assumes constant scattering properties. The modified Beer-Lambert law that includes a differential path length factor to account for scattering within the tissue can be used to convert measurements of light attenuation into concentrations of these chromophores. The Beer-Lambert law is defined as:
Δ A = L ⋅ Δ μ a
where A = light attenuation, L = differential path length, and µa = absorption scattering coefficient.
This calculation is very reliable when applied to an infant’s head because the skull is thin enough to allow transillumination of light from one side to the other. In an adult, however, the relative thickness of the scalp, skull, and brain prevents transmission spectroscopy, and NIRS must be used in reflectance mode, with the emitting and receiving optodes placed on the same side of the head. Reflectance mode oximetry depends on a proportion of the light that passes through brain tissue. The human head is comprised of multiple tissue layers, all of which have different scattering properties and contain varying concentrations of light-absorbing compounds. This results in the introduction of unknown, nonlinear variables for light absorption and scattering coefficients. Various algorithms have been applied to attempt quantification, but doubts are expressed over their accuracy.
Extracranial Contamination
Extracranial contamination is a major issue during the use of NIRS in the adult brain, although extracranial contributions to NIRS measurements initially were thought to be insignificant. Bone was considered to be transparent to light, whereas the skin contribution was estimated to be approximately 5%. Over time, however, numerous studies have demonstrated that extracranial contamination is a significant problem.
In reflectance mode, the near infrared light emitter and detectors are placed within a few centimeters of each other on the surface of the scalp. The larger the optode distance used, the greater the proportion of near infrared light that should pass through the brain tissue. However, even when a large inter-optode distance is used there is still a major contribution from the extracranial tissue to the NIRS signal. Other methods are used to correct for the effects of the extracranial tissue layers; one is to subtract measurements from two receiving optodes placed in line with the transmitting optode. The underlying assumption is that the path length of light through the extracranial tissues is the same for both of the receiving probes. The difference in absorption coefficients between the two probes should then reflect absorption and scatter within the cerebral tissue alone. However, this is an oversimplification that has resulted in measurement inaccuracies.
The concepts about light distribution in the adult head are complex and are still being investigated. Mathematical in vitro modeling has attempted to predict the distribution of light through the extracerebral layers. Multilayer studies have highlighted the influence that superficial tissue layers can have on light distribution in tissues and provide further evidence that NIRS measurements may underestimate changes in concentration. Mathematical simulations using Monte Carlo techniques and finite element modeling have demonstrated that the cerebrospinal fluid (CSF) layer that surrounds the brain can act as an optical short-cut for light as it passes through the tissues. It has been suggested that this phenomenon can prevent the near infrared light from penetrating any deeper than the cerebral grey matter, or even cause it to bypass the cerebral compartment altogether.
Modern near infrared spectrometers detect oxygenation changes in cerebral tissues. However, many machines still remain sensitive, by varying degrees, to changes in extracerebral oxygenation. These changes can influence estimates of cerebral oxygenation. Conditions in which changes in extracerebral oxygenation may seem unlikely should not be taken for granted. For example, changes in extracerebral oxygenation in the scalp have been detected during the performance of functional activation tasks. Even in the absence of extracerebral oxygenation changes, the extracerebral tissues may confound attempts to calibrate and quantify changes in cerebral oxygenation. In any NIRS study it is essential to consider whether the changes detected could be due, even in part, to extracerebral contamination. To reliably measure cerebral oxygenation with NIRS, extracerebral changes must be shown to be insignificant, or their effect eliminated.
Instrumentation
There has been rapid growth and development of NIRS technology, and novel optical instruments continue to be developed and evaluated for diagnostic and functional imaging in the clinical environment. Although the early machines were useful only as trend monitors, current commercial machines detect changes in optical attenuation of a number of wavelengths of light, are compact, are portable, and enable noninvasive measurements of cerebral oxygenation at the bedside.
The INVOS Cerebral Oximeter (Somanetics, Troy, MI) and the Hamamatsu 100, 200, and 300 (Hamamatsu Photonics KK, Hamamatsu, Japan) are among popular commercially available devices for brain monitoring. In addition, multiprobe devices (e.g., INVOS 5100) are used in newborns or pediatric intensive care units (ICUs) to examine regional cerebral (rSO 2 C), splanchnic (rSO 2 S), and renal (rSO 2 R) tissue oxygenation. Several research groups also have produced their own specialized in-house NIRS equipment, which may be available locally. When published values from different instruments are compared, it should be remembered that different instruments may provide different values for cerebral tissue oxygen saturations because the data are derived from different volumes of tissue. Among current techniques, spatially resolved spectroscopy appears to be the most promising, and reliably shows changes in chromophores in brain tissue.
Spatially Resolved Spectroscopy
Spatially resolved spectroscopy is described in a number of publications. Spatial resolution relies on the measurement of the attenuation gradient as a function of source-detector separation. Using a modified diffusion equation, a product of the absorption and scattering coefficients is calculated. When the tissue is treated as homogeneous, the scattering coefficient can be assumed to be a constant (k) in the near infrared wavelength. To increase the accuracy of the calculation, however, a wavelength dependency for the scattering coefficient is derived in the form of:
k ( 1 − h λ )
where λ = wavelength and h is the normalized slope of the scattering coefficient along λ. From here, the relative absorption coefficients and thus the relative concentrations of HbO 2 and HHb can be obtained.
Hamamatsu NIRO 300
The NIRO 300 is a noninvasive bedside monitor that provides continuous online measurements of hemoglobin and cytochrome oxidase concentrations, and a calculated tissue oxygen index (TOI). The optodes are held in a black custom-made holder that allows them to be set at either a 4.5- or 5-cm distance. By using two sets of optodes, two channel simultaneous measurements are possible.
Four wavelengths of light (775, 810, 850, and 910 nm) are delivered by four pulsed laser diodes, and the scattered light is detected by three closely placed photodiodes. The concentration changes of the chromophores HbO 2 , HHb, total hemoglobin, and cytochrome oxidase are measured by conventional differential spectroscopy using a modified Beer-Lambert law, whereas the basic principle behind calculation of the tissue oxygenation index is spatially resolved reflectance spectroscopy. The basic measurement made is the rate of increase of light attenuation with respect to source or detector spacing, and TOI is calculated from this using photon diffusion theory. The hemoglobin and cytochrome concentration changes are measured by the middle photodiode, whereas TOI is measured by using all three. TOI is the ratio of oxygenated to total tissue hemoglobin and can be expressed as:
HbO 2 HbO 2 + HHb × 100
The NIRO 200 and 100 are the latest commercial updates to the NIRO 300 and use only three wavelengths of delivered light (775, 810, and 850 nm) for the measurement calculations. The NIRO 200 is a two-channel system that allows bihemispheric data to be collected, whereas the NIRO 100 is a single-channel machine.
Clinical Neuromonitoring
Clinical Application
NIRS has many applications in the ICU and the operating room, and it can be used to detect real-time changes in regional oxygen saturation of cerebral and somatic tissues. Its use is well described in cardiac surgery and neonatal and pediatric critical care, and most recently several studies have described use of NIRS to evaluate tissue perfusion and the dynamic response of the microcirculation to a stress test in patients who are dehydrated, septic, or in shock. These studies in critically ill adults suggest NIRS can be used to guide goal-directed therapy and that blood pressure is not always a reliable surrogate for peripheral blood flow and oxygen delivery. In addition, greater mortality is observed in septic patients in whom forearm skeletal muscle rSO 2 is less than or equal to 60% throughout the first 24 hours of ICU care and low tissue oxygen saturation (StO 2 ) persists in sicker patients (e.g., greater Acute Physiology and Chronic Health Evaluation [APACHE] or Sequential Organ Failure Assessment [SOFA] scores). This information about systemic oxygenation can be very useful in many patients in the neurocritical care unit (NCCU) who may develop sepsis or systemic inflammatory response syndrome (SIRS) among other systemic disorders, because many shock states have been shown to be associated with a decrease in perfused capillary density and an increase in the heterogeneity of microcirculatory perfusion.
The ability to monitor cerebral oxygenation noninvasively and at the bedside in patients at risk of cerebral ischemia is a potential advantage of NIRS and its use to evaluate changes in regional cerebral oxygenation, cerebral blood flow (CBF), and oxygen utilization in the brain is reported in a variety of medical and neurosurgical conditions, in particular those associated with disturbed cerebral circulation such as head injury and intracranial hemorrhage, and in patients undergoing carotid endarterectomy. In head-injured patients changes in HbO 2 detected by NIRS appear to correlate well with changes in SjvO 2 , TCD, and laser Doppler flowmetry (LDF). The information for NIRS also appears to have some prognostic information (e.g., cerebral oxygen desaturation is associated with prolonged intensive care and total hospital lengths of stay). Zweifel et al. compared an NIRS-based index of cerebrovascular pressure reactivity (PRx), called total hemoglobin reactivity (THx) , against standard measurements of PRx that requires invasive intracranial pressure (ICP) monitoring. PRx and THx had a significant association across averaged individual recordings and across patients. Optimal cerebral perfusion pressure (CPP) could also be assessed with THx in about half of patients. These data suggest that NIRS may be useful in some traumatic brain injury (TBI) patients to optimize and target therapy particularly those who do not have an ICP monitor. Others have described the use of NIRS to help guide therapy for vasospasm after subarachnoid hemorrhage (SAH) and observed that NIRS may distinguish different cerebral oxygenation patterns associated with different seizure types.
Intraoperative use of NIRS during cardiac surgery or carotid endarterectomy (CEA) is well described. These studies have helped to define a potential NIRS-based “threshold” for ischemia particularly when using spatially resolved spectroscopy (e.g., NIRO 300, Hamamatsu Photonics). However, it is difficult to determine how useful NIRS is during cardiac surgery without fully understanding how extracorporeal circulation affects the assumptions on which NIRS technology is based. The information from CEA studies may be more relevant to the NCCU. For example, the NIRO 500 can be used successfully to monitor patients who undergo CEA under general anesthesia. In some studies once the extracranial component is subtracted, a threshold for severe critical ischemia can be defined. This threshold then can be used to guide the selective use of an intraoperative carotid artery shunt. Other studies using bilateral cerebral oximetry in patients undergoing CEA under regional anesthesia or when INVOS is compared with somatosensory evoked potentials ; however, they have not found it possible to determine the critical cerebral oxygen saturation or change in saturation that requires shunt insertion because of marked individual variability of rSO 2 and the derived changes. In addition, cerebral oxygen saturation may decrease without neurologic dysfunction.
Troubleshooting
Troubleshooting advice provided by the manufacturer comes with all near infrared spectrometers and in the first instance the user should consult the appropriate operating guide. However, some issues may be considered common to all NIRS devices and studies.
First, the light source and detector optodes should be spaced according to the study requirements, although increasing the optode distance may help improve the sensitivity of NIRS to brain tissue changes. An optode distance greater than 3 to 4 cm should reduce the extracerebral contribution because as the optode distance is increased, a greater proportion of light passes through the brain. Germon et al. in a study in which optode distance was increased during NIRS studies suggest that a separation of 5.5 cm may increase sensitivity to cerebral changes, whereas extracranial effects may plateau at 4.8 to 5.5 cm. By contrast when placed close together most of the transmitted light likely passes through the scalp and superficial tissue. For example, in normal volunteers during verbal fluency tasks, optodes placed 5 mm apart may only measure skin changes rather than reflect any cerebral function. The distance between optodes and emitter also may depend on practical constraints such as other monitors, surgical sites, dressings, and the signal-to-noise ratio. Measurement of both arterial blood pressure and peripheral saturation can address in part how extracranial contamination affects the signal. Direct monitoring of skin flow is possible using LDF to assess relative changes in scalp blood flow. If the LDF probe is placed on the skin in the immediate vicinity of the NIRS probes, skin flow changes that may affect NIRS measurements can be crudely monitored.
Second, most NIRS systems are placed in the frontotemporal region for adult brain tissue monitoring. For optimal performance the skin surface should be clean and dry and the optodes placed high on the forehead and in a position to avoid the sagittal sinus, muscle (temporalis muscle), and midline. Hair also should be avoided whenever possible because it can reduce return of the near infrared signal because of light absorption by the hair follicles. When hair is present it also is difficult to adequately seal out light. Third, ambient light should be eliminated. The use of a bandage to eliminate light that covers the optode holder is beneficial. It also will help to reduce scalp movement during the study. Care is needed to avoid tissue ischemia and necrosis especially with long-term monitoring. Fourth, it is useful to support the optical fiber cables to prevent both damage and the weight of the fibers dislodging the optodes. Finally, using NIRS as part of a multimodal monitoring system helps to confirm true clinical changes versus artifact. When interpreting NIRS values, signal change or percentage change from baseline may be more useful than absolute values. This is particularly true if comparisons between individual patients, clinical centers, or NIRS machines are required.
Limitations
A number of limitations should be considered before NIRS can contribute to clinical decision making. First, the major limitation of NIRS is extracranial contamination. The heterogeneity of the scalp and skull affect the transmission of near infrared light through the head and may cause significant individual variability. Second, NIRS monitors cerebral oxygen saturation in an uncertain mixture of arteries, capillaries, and veins. The sampling volume of gray and white matter is unknown. Third, current NIRS probes are not magnetic resonance imaging (MRI) compatible; this limits its use in potential MRI validation studies. Fourth, NIRS does not resolve focal abnormalities in CBF but may be useful as a monitor of general changes that indicate secondary brain injury. Fifth, some early studies suggest elevated intracranial pressure may limit the reliability of NIRS. Finally, the quantitative accuracy of calculated cerebral oxygen saturation still needs to be established, and a range of normal values defined.
Evaluation of the NIRO 300 as a Monitor of Cerebral Ischemia
Patients who undergo CEA often may experience brief periods of cerebral ischemia during cross-clamping of the internal carotid artery (ICA). During CEA, segregation of the NIRS signal between intra- and extracranial vascular territories is possible by staged application of vascular clamps to the external and internal carotid arteries. In this way, the authors have previously identified NIRS thresholds for cerebral ischemia by extracting the extracranial signal.
At the authors’ institution routine multimodal monitoring during CEA includes frontal cutaneous LDF, TCD mean flow velocity (FV) measurements of the ipsilateral middle cerebral artery, and mean arterial blood pressure (ABP). The LDF (Moor Instruments Ltd, Axminster, UK) is modified to use light in the visible spectrum (wavelength 650 nm) to avoid any interference with the NIRS signal. The authors incorporated the NIRO 300 into this multimodal monitoring system to detect cerebral hemodynamic changes during highly controlled conditions and determine the anatomic source of the calculated TOI (%) ( Fig. 33.2 ).
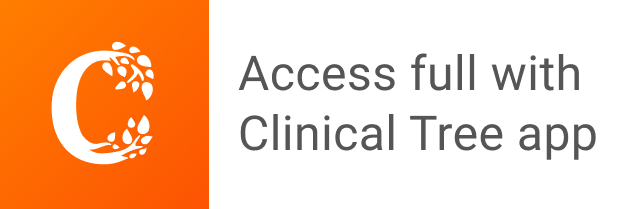