Figure 78.1. Area of restricted diffusion in the left superior frontal gyrus shown in a patient with right leg clonic status epilepticus. (From Wieshmann UC, Symms MR, Shorvon SD. Diffusion changes in status epilepticus. Lancet. 1997;350(9076):493–494, with permission.)
Following this case report, multiple systematic investigations have explored peri-ictal DWI in an attempt to assess the usefulness of this novel technology to delineate the ictal-onset zone. Overall, the presence of dynamic diffusion changes has been documented in the majority of cases, but the correlation between the presumed epileptogenic zone and the diffusion changes is quite variable (31–35). Correlations seem closer in patients with longer seizures (or status) and short duration between seizure end and scan (31,33). A single case report in man confirms that restricted diffusion is a marker of the ictal-onset zone: An area of restricted diffusion adjacent to the lesion in the right frontal lobe in a patient with repetitive prolonged focal motor seizures corresponded to the region of focal electrocorticographic seizures that was mapped intraoperatively (36).
Studies using DTI to study peri-ictal changes allowed for comparison of the sensitivity of diffusivity changes versus anisotropy changes and to assess whether DTI provides higher sensitivity to seizure-induced changes. The results remain rather disappointing, and it has become apparent that dynamic changes affected the diffusivity to a much higher degree than the directionality (32). Peri-ictal mean diffusivity reductions are seen in about half of the patients investigated, but only a relatively small proportion (20%) colocalized with the presumed ictal-onset zone, even when patients were scanned within 45 minutes after the seizure (35). In addition, whole-brain analysis using statistical parametric mapping (SPM) revealed distant areas of diffusivity change, possibly highlighting the network involved in ictal spread.
In order to minimize delays between seizure and scanning, flumazenil was used to induce seizures in patients assessed for epilepsy surgery (37). Despite almost immediate MRI scanning, diffusivity decreases were seen in the hippocampus on the seizure-onset side and in both parahippocampal gyri.
Therefore, it seems possible that diffusion changes after single seizures appear more transient and require immediate access to scanning. If in the future such an environment can be provided, in combination with higher-resolution scanning and possibly also higher field strengths of MR scanners, postictal studies may be of higher yield.
Interictal DTI and DWI Changes
Temporal Lobe Epilepsy
Patients with mesial temporal lobe epilepsy (TLE) due to hippocampal sclerosis reveal increased diffusivity in the ipsilateral hippocampus, indicative of structural disorganization and expansion of extracellular space, reflecting neuronal loss and other microstructural changes (38–43). These changes parallel the abnormalities noted on conventional MRI scans with atrophy and T2 signal increase. When assessing DWI compared to conventional MRI using volumetric T1 acquisitions and FLAIR, it was not more sensitive in detecting hippocampal sclerosis (40). In addition, in patients without lateralizing differences between the hippocampal formations, both hippocampi often showed increased apparent diffusion coefficient compared to a control population, indicating bilaterality of the disease. Such bilateral abnormalities are present throughout the limbic system, including fornix and cingulum in both adults (44,45) and children (46).
When patients with TLE were evaluated using a region-of-interest approach, diffusion abnormalities extend into the ipsilateral hemisphere and even into the contralateral hemisphere (44,45,47–50), as has recently been summarized in a meta-analysis of 13 studies comparing white matter tracts ipsilateral and contralateral to the epileptogenic temporal lobe to controls (51). Such more widespread changes have been confirmed using voxel-based approaches, which compare one individual to a group of normal controls and thus do not have selection bias to a particular region of interest (50). These changes are not reversible after successful temporal lobectomy, which may suggest structural abnormalities as opposed to functional changes due to seizures (52).
DTI can also identify abnormal areas in temporal and extratemporal focal epilepsy with normal conventional MRI. Out of 30 patients, increases in diffusivity were found in 8 patients (26%), and 6 of the 8 diffusivity alterations were in the presumed epileptogenic zone (53). In addition, group analysis of nonlesional left TLE patients revealed increased diffusivity and reduced anisotropy within the ipsilateral temporal lobe; the right TLE group displayed a trend in the same direction (53). Although such a group effect is not helpful in an individual patient, it suggests that given greater sensitivity and increased signal-to-noise ratios, an effect in individual patients may be demonstrated. Overall, such occult lesions are most likely caused by disruption of white matter architecture due to dysgenesis or by seizure- related damage leading to atrophy, gliosis, and expansion of the extracellular space, resulting in increased diffusivity and potentially also decreased anisotropy.
Extratemporal Lobe Epilepsy
Case numbers of extratemporal epilepsies in the presurgical epilepsy workup are rising, and these cases are often challenging as precise localization of the epileptogenic zone in relation to cortical function is mandatory. Diffusion changes are seen in a variety of lesions associated with focal epilepsy and often localize outside the temporal lobe, such as cortical dysplasia.
Reductions in anisotropy and increase in diffusivity within the MRI visible lesion and also outside of it have been reported in a variety of cortical dysplasias (54,55). Reductions in FA in most patients in normal-appearing white matter surrounding dysplastic lesions are likely due to gliosis, axonal loss, poor myelination, or increased cell bodies (e.g., ectopic or abnormal neurons, balloon cells). In addition, distant anisotropic changes can also be observed, possibly due to wallerian degeneration of tracts or gliosis resulting from chronic seizures. Investigations on the impact of cortical dysplasia on connectivity and adjacent tracts showed decreased tract size and displacement of tracts in larger dysplasias, as well as rarefaction of subcortical connections surrounding cortical dysplasia (56). Figure 78.2 shows altered connectivity in a patient with right temporooccipital epilepsy, with polymicrogyria and heterotopic gray matter in the same region.
Figure 78.2. Malformations of cortical development and alterations of connectivity. Twenty-six-year-old with intractable focal epilepsy arising from the right temporooccipital region. MRI showed right > left posterior quadrant polymicrogyria and heterotopic gray matter in the right posterior quadrant. A: Axial colorized fiber orientation maps showing displacement of the right superior frontooccipital fasciculus and superior longitudinal fasciculus. B: Two-dimensional illustration of the tractography results overlaid onto the T1 image demonstrates the spatial relationship between the heterotopic gray matter and the white matter tracts.
Probing Diffusion Changes: What Can It Tell Us in Human Epilepsy
Analyzing the pattern of diffusion changes with respect to diffusivities parallel and perpendicular (radial) to the main axonal direction provides in vivo insights into the underlying cause of diffusivity increase and decreased FA. Several studies revealed that the most commonly seen pattern of DTI changes associated with focal epilepsy was unchanged parallel diffusivity and increased perpendicular diffusivity (45,47,48,57,58). As detailed above, such a pattern of FA changes seen in most studies evaluating DTI in TLE is most consistent with chronic wallerian degeneration, possibly due to cell loss in the temporal lobe secondary to seizure-induced cell death.
In order to evaluate potential mechanisms for such more widespread diffusion changes in TLE, it was investigated if different underlying pathologies as determined by preoperative MRI cause differential diffusion changes (45). Patients with TLE and hippocampal sclerosis were compared to nonlesional TLE: While some white matter bundles are affected equally in both forms of TLE, abnormalities of the bundles directly related to the mesial temporal structures (i.e., the fornix and cingulum) appear to be unique to TLE with hippocampal sclerosis.
It has been demonstrated that DTI can be used to delineate the neurocognitive correlates of localized white matter damage in TLE, such as working memory (59), visual and verbal memory, and language dysfunction (57,60), and research into such structure function relationships is ongoing.
Interictal DTI and DWI Changes: Conclusion
Interictal DTI highlights areas of abnormal diffusion measures in temporal and extratemporal lobe epilepsies, both lesional and nonlesional. Specifically, mean diffusivity appears more sensitive to changes seen in patients with chronic refractory epilepsy compared to FA. The only exception may be cortical dysplasias. DTI abnormalities are seen in all areas, indicating pathology on conventional MRI. In addition, DTI changes may often be found outside the lesions, both contiguous and less frequently also noncontiguous to the lesion. Abnormalities mostly with increased diffusivity and reduced FA have also been found in patients with cryptogenic focal epilepsy. Analysis of water diffusivity changes reveals a pattern of increase in perpendicular diffusivity and not of parallel diffusivity. This may indicate wallerian degeneration as one of the main mechanisms accounting for the structural changes underlying the DTI abnormalities remote from focus and lesion.
Such abnormal areas in patients with intractable epilepsy, therefore, probably represent structural disruption, possibly reflecting either an underlying pathology or gliosis due to secondary damage. This requires further study with MRI–histology correlation in more patients.
Interictal DTI and Irritative and Ictal-Onset Zone
Close correlations between the interictal abnormalities highlighted using DTI, pathology, and epileptogenicity are rare. Intracranial recordings in a patient with cryptogenic focal epilepsy showed seizure onset in the right orbitofrontal region, colocalizing with an area of abnormal diffusivity (61), and postresection pathology revealed gliosis. Of note, however, is that this patient is not completely seizure free.
Few papers have evaluated in detail the concordance between diffusion abnormalities and irritative zone and ictal-onset zone as evaluated using invasive recordings. Two studies have used voxel-based statistical approaches to highlight areas of abnormal diffusion in a small number of patients undergoing stereo-EEG evaluations (62,63). In one study (63), 13 of the 16 patients were found to have DTI abnormalities. DTI abnormalities consisted mainly of increases in mean diffusivity and were concordant with the epileptogenic zone in seven. FA abnormalities added little in localization. The specificity of DTI abnormalities was better in extratemporal lobe epilepsy: 20% of TLE had congruent findings, whereas four of five extratemporal epilepsies concurred.
Another study investigated 14 patients with frontal lobe epilepsy (9 nonlesional), and almost all patients showed areas of increased diffusivity (62). In this study, the sensitivity of diffusion imaging in defining regions that were the site of electrical abnormalities was about 57% for the area of seizure onset and 65% for the irritative zone, and the specificity was low. It is of note, however, that areas of diffusion abnormalities may not have been sampled, as coverage is necessarily limited with stereo-EEG. An interesting aspect in this study is that lesional epilepsies had very high sensitivity, as the lesion led to diffusion abnormalities, but very low specificity. In nonlesional epilepsies, cases in which epileptologists may particularly turn to novel imaging for additional support of a hypothesis for invasive recordings, three out of the nine patients had diffusion changes in the seizure-onset zone.
Overall, the limited data available suggest that diffusion changes correlate better with areas of interictal spiking than the ictal onset. Furthermore, the presence of DTI abnormalities certainly does not mean that the seizures are arising in the vicinity. However, DTI changes may provide some additional information to guide placement of invasive electrodes. Interestingly, a recent study using a surface-based laminar analysis of gray and white matter showed differential findings regarding diffusivity in the seizure-onset zone (64), compared to healthy controls. In 18 children with nonlesion extratemporal lobe epilepsy, such analysis revealed increased diffusivity in the cortical gray matter, most pronounced in the outer fraction of the gray matter, also involving the white matter underlying the epileptic cortex. The electrographically normal cortex, in contrast, showed decreased diffusivity in inner and middle cortical fractions compared to controls. The authors speculated whether this may be indicative of surround inhibition.
In conclusion, data remain sparse and correlating electroclinical abnormalities using invasive recordings with diffusion changes may allow for better insights in the future.
Tractography and Epilepsy Surgery
DTI is the first imaging modality that allows direct noninvasive visualization of white matter tracts. Several investigations have focused on retrospectively correlating DTI-based tractography with postoperative deficits, to assess if this technology could provide predictive information for a deficit and could maybe even aid in preservation of function if such information were integrated in neuronavigation systems. Anterior temporal lobectomies can cause a contralateral superior quadrantanopsia in up to 10% of patients by disrupting Meyer loop. The anterior extent of Meyer loop has large interindividual variability and cannot be visualized using conventional imaging (65). Tractography has been used to demonstrate the optic radiation in normal subjects (66), and its use was subsequently explored for temporal lobectomies (67,68) and the anteroposterior extent of the damage to Meyer loop as determined postoperatively correlated well with the degree of visual field loss (68). Pre- and intraoperative DTI-based fiber tracking (69) showed significant correlation between the fiber tracking estimation and the outcome of visual field deficits after surgery.
These data provide evidence that tractography has the potential to inform about risks of epilepsy surgery procedures. Once successfully implemented into neuronavigation systems, this information may also be used intraoperatively to tailor resections (70). Aside from the technical issues of performing tractography in health and disease, the intraoperative brain shift after craniotomy is another significant impediment. The availability of intraoperative MRI may represent one method to correct for this movement and may improve the accuracy of the data to aid surgical planning.
Extratemporal surgeries will also benefit from visualizing crucial connections and tracts such as the pyramidal tract. Implementation of DTI-based tractography has already been shown to benefit patients undergoing brain tumor surgeries and resections of vascular malformations (70–74) and will certainly be increasingly used in epilepsy surgery.
EEG–fMRI
Up to now, the localization of the generators of interictal epileptiform discharges (IEDs) has been mainly addressed through EEG and magnetoencephalography (MEG), techniques with exquisite temporal resolution. fMRI offers good spatial resolution and localization of events that are accompanied by a blood oxygen level–dependent (BOLD) response (5). A question that must be addressed first when considering fMRI as an epilepsy localization tool is whether epileptiform paroxysmal events (interictal and ictal) are associated with detectable BOLD signal changes. In this regard, evidence from multiple sources including visual observation of the cortex during surgery, PET, SPECT, and near-infrared spectroscopy (NIRS) is conclusive: Seizures are commonly accompanied by regional hemodynamic changes. In the case of interictal discharges, the above techniques are unsuitable, some due to their fundamentally limited temporal resolution (PET, SPECT) and others due to limited spatial sensitivity profiles, such as NIRS. fMRI with its temporal resolution of the order of a few seconds and excellent whole-brain mapping capability may offer a way to map out hemodynamic changes throughout the brain linked to short events from individual IEDs, to runs of spikes, and sharp waves to seizures. Importantly, fMRI’s localization capability is independent of the extent or complexity of the involved brain region or regions, in contrast to EEG/MEG source analysis, and therefore may be more capable of revealing their full extent. The EEG is used as an indicator of events of interest, such as spikes or ictal discharges, from which a model of the fMRI signal is derived a posteriori and used for analysis of the fMRI time series data. The adjunct of simultaneous video can provide important information for the fMRI modeling (75).
fMRI of Spontaneous Brain Activity: Data Acquisition, Analysis, and Interpretation
In the following paragraph, we discuss some of the main technical aspects, applications, and findings of fMRI used to map spontaneous hemodynamic changes in patients with epilepsy. In most studies, the patient is asked to lie in the scanner with eyes closed and whole-brain scanning is performed in the expectation of capturing events of interest, usually interictal discharges, although drugs have been used in a small number of studies to modulate epileptiform activity specifically for the purpose of fMRI (76,77). Because of the random nature and temporal characteristics of epileptic activity, the fMRI data thus acquired are commonly analyzed within the framework of event-related designs, in contrast to the more conventional block designs used in many cognitive studies. This is done using the EEG (and video, when available) as a basis for modeling the variations in the BOLD signal related to epileptic activity. For this purpose, short epileptiform discharges such as single spikes have been likened to, and represented mathematically as, brief stimuli. As described in Chapter 79, the BOLD response to brief stimuli develops and resolves over a period of roughly 25 seconds, generally peaking between 5 and 7 seconds, and then undershoots the baseline slightly, roughly 15 seconds poststimulus, before returning to baseline, and is therefore essentially biphasic (i.e., it has positive and negative phases); this is the hemodynamic response function (HRF) (78). We note that a substantial amount of intersubject and interregional variability of the HRF in healthy subjects has been documented (79). The possibility of deviation of the shape of the HRF in patients with epilepsy due to the effects of pathology or other factors has implications for the technique’s sensitivity and potential clinical usefulness. Furthermore, the choice of an accurate representation of the HRF is greater for the detection of regions of BOLD change in event-related designs than for block designs. Importantly, event-related designs are generally less efficient than block designs for the detection of BOLD changes.
Before embarking on a review of the application of EEG–fMRI in focal epilepsy, we discuss a small number of fMRI studies of seizures for which concurrent EEG was not used.
fMRI of Seizures (Without Concurrent EEG)
fMRI data acquired in the resting state have been used to map BOLD signal changes linked to ictal or peri-ictal events. The lack of concurrent EEG means that the analysis and interpretation of the fMRI is heavily reliant on clinical changes observed during the events and the use of this information for the labeling of scans as being ictal or interictal. Apparent BOLD changes were revealed by subtraction of scans acquired at baseline from scans acquired during motor seizures in a child (80). In one study (81), the authors identified patterns of signal change in the absence of any overt ictal activity, and these changes were consistent with invasive localization. In another case report, ictal signs identified in relation to scan acquisition times were used to plot T2* signal changes relative to a baseline value voxel by voxel, revealing regions of signal increase and decrease preceding and during the motor seizure (82). Federico et al. (83) studied preictal fMRI changes based on visual observation without concurrent EEG in two cases. Regions of signal change were identified by comparing blocks of scans immediately preceding the seizures to blocks acquired 3 to 5 minutes before ictal onset. Inspection of the signal time course in those regions and “control” regions revealed patterns suggestive of specific preictal BOLD increases and decreases to a lesser extent taking place around 10 minutes before seizure onset in one case, similar to a case in which EEG was recorded during fMRI. Although interesting, this type of analysis of fMRI data is suboptimal in many respects such as potential subjectivity of the identification of the event onset (and the resulting model of the BOLD signal) and possible bias associated with physiologic and scanner-related artifacts (5).
EEG-Correlated fMRI: The Technique
While the clinical manifestations may be used as event markers for seizures, this is not the case for subclinical events such as IEDs. Therefore, the study of the hemodynamic changes associated with IED necessitates the recording of EEG during fMRI. Concurrent EEG, and video, may help in the interpretation of ictal events for the purpose of fMRI modeling. The technique that consists in the simultaneous acquisition of EEG and fMRI data is commonly referred to as EEG-correlated fMRI, or EEG–fMRI. Due to the various interactions between the two technologies, data quality and patient safety are serious concerns and have been the subject of a large amount of work since the first ever EEG recording took place inside an MR scanner (84). The main problems are pulse-related and image acquisition artifacts on EEG, image artifacts due to passive components (EEG leads and electrodes) and active components (EEG amplifier/digitizer) (85).
While many developments have led to the commercialization of EEG recording systems capable of providing the investigator with basic tools capable of producing good quality data for some applications, such as EEG–fMRI of visually identifiable IED, EEG and image data quality continue to preoccupy many users partly because some problems may never be completely solved (e.g., pulse-related artifact on EEG) and partly because the application boundaries continue to be pushed (increasing scanner field strength, study of ever more subtle EEG features, etc.). Therefore, EEG–fMRI data acquisition continues to be a field of development and investigators undertaking EEG–fMRI investigations in epilepsy are advised to obtain continuous technical support.
EEG–fMRI of Seizures
Although potentially most relevant for presurgical localization, the study of the hemodynamic changes during ictal patterns is problematic due to safety concerns, and the rarity and unpredictability of seizures in most patients mean that ictal EEG–fMRI studies are generally fortuitous. Once such data have been acquired, their analysis is made difficult due to the effects of head motion, the long duration of the events (same time scale as some fMRI artifacts), and the uncertain relationship between clinical and EEG manifestations on one hand and the pathologic neurophysiologic activity on the other. Nonetheless, based on simple fMRI modeling assumptions, ictal EEG–fMRI has shown BOLD increases often of larger amplitude and extent than interictal patterns and associated higher yield* than interictal EEG–fMRI. For example, using a flexible modeling strategy adapted to long events, a large, electroclinically concordant BOLD increase was revealed in relation to a single subclinical seizure (86). In a patient with multiple seizures, a similarly concordant pattern of BOLD increase was revealed with large contralateral areas of simultaneous BOLD decreases (87). In a series of eight selected cases with malformations of cortical development (MCD) in whom seizures were studied using EEG–fMRI, the relationship between ictal and interictal BOLD patterns and MR-visible lesions seemed to reflect the specific pathology; for example, in cases with nodular heterotopia, there was a tendency for the ictal BOLD changes to involve the overlying cortex (88). In a recent series of patients recruited specifically for the purpose of studying preictal and ictal hemodynamics, typical seizures were captured in 20/55 cases (89). Across the group, there was a tendency for widespread preictal fMRI changes, followed by more localized, often in the vicinity of the confirmed or presumed seizure-onset zone, hemodynamic changes at onset and subsequent spread during seizure evolution.
* Yield: proportion of cases in which significant event-related BOLD changes are revealed.
† The actual delay may have varied due to the manual nature of the process, but an uncertainty of the order of ±1 second would be of little consequence given the time scale of BOLD changes.
EEG–fMRI of Interictal Epileptiform Discharges
The overwhelming majority of cases studied using EEG–fMRI have focused on revealing BOLD changes linked to IED. The primary aim of early studies in patients with focal epilepsy has been the demonstration of IED-related changes to localize the generators of the discharges, with the focus gradually shifting to the study of the details of the BOLD map localization in relation to other tests (MRI, EEG, etc.) and other aspects of these patterns such as the sign of BOLD change.
Two variants of the technique have been used for this purpose: IED-triggered fMRI and the more flexible and now widely used continuous EEG–fMRI. In IED-triggered fMRI, the MR acquisition, usually of a single image volume, was started following the identification of a spike or sharp wave; a fixed delay of a few seconds between spike and scan was employed, calculated based on the assumption that IED-related BOLD change will follow the course of the normal HRF.† A set of images acquired following IED were compared to images acquired following periods of background EEG. Such studies revealed significant BOLD increases in a large proportion of cases mostly concordant with the presumed or suspected generator localization (76,77,90–94). We note that these studies largely ignored the possibility of IED-related BOLD decreases. The finding of BOLD increases in expected locations in the majority of cases in which IED were captured confers a degree of validity to the assumption that IED-related changes are roughly in line with the HRF derived from physiologic stimuli in healthy subjects, peaking at around 6 seconds postspike.
Due to the appeal of having access to the entire EEG record during scanning, IED-triggered fMRI has now been superseded by continuous EEG–fMRI that was made possible by EEG scanning-related artifact correction algorithms (95,96). In continuous EEG–fMRI, scans are acquired without interruption resulting in a continuous time series of scan data. Importantly, the analysis of continuous EEG–fMRI data to reveal regions of increase or decrease BOLD signal related to events of interest (e.g., pathologic EEG discharges) is based on building models (general linear model [GLM]) of the BOLD signal over the entire scanning session, which can be challenging due to spontaneous changes in brain state at rest (97,98).
The analysis of EEG–fMRI is commonly based on conventional, visual EEG interpretation by expert observers and therefore suffers from the same limitations, although the impact of this subjectivity has not been thoroughly investigated (see (5) for a review of the technique’s principles and limitations; see (99) for a rare study on the impact of EEG interpretation on the fMRI results). Using this approach in selected case series in focal epilepsy, and assuming that the IED-related HRF does not deviate substantially from the norm, regions of statistically significant BOLD signal changes were revealed in around 70% of the cases in whom IEDs were captured during scanning sessions with durations of the order of 40 to 60 minutes (90,100). Overall, the pattern of BOLD increases and decreases is often complex, although the localization of the BOLD increases tends to match the presumed or confirmed IED generator localization. BOLD decreases tend to be more remote and less representative of the IED field distribution (100,101). In TLE, a similar mix of BOLD increases and decreases involving the ipsilateral and contralateral (homologous) cortex was observed (100–102), with a consistent pattern of BOLD decrease in the precuneus (103), reminiscent of the so-called default-mode network (Fig. 78.3) (104).
Figure 78.3. IED-related BOLD changes. Patient with left hippocampal sclerosis and left temporal IEDs who underwent EEG–fMRI. A: BOLD increase in the left temporal lobe with smaller clusters in the occipital region. The red arrow and cross hair represent the location of the most significant BOLD change. The left of the figure shows all statistically significant BOLD changes superimposed onto the SPM “glass brain.” The right of the figure shows parasagittal, coronal, and axial sections through the EPI data at the location of the statistical maximum. B: Retrosplenial BOLD decrease associated with the same IED. This effect, which is thought not to represent activity of the source of the spike seen on EEG, is commonly observed in relation to interictal activity in the temporal lobe. (From Salek-Haddadi A, Diehl B, Hamandi K, et al. Hemodynamic correlates of epileptiform discharges: an EEG-fMRI study of 63 patients with focal epilepsy. Brain Res. 2006;1088(1):148–166, with permission.)
One of the advantages of continuous EEG–fMRI over spike-triggered fMRI is that it allows one to estimate the shape of the IED-related HRF, which is important if deviations from the norm are suspected with potential impact for analysis sensitivity. This can be done by using different sets of functions instead of the canonical HRF such as Fourier expansions or series of gamma functions (105–107
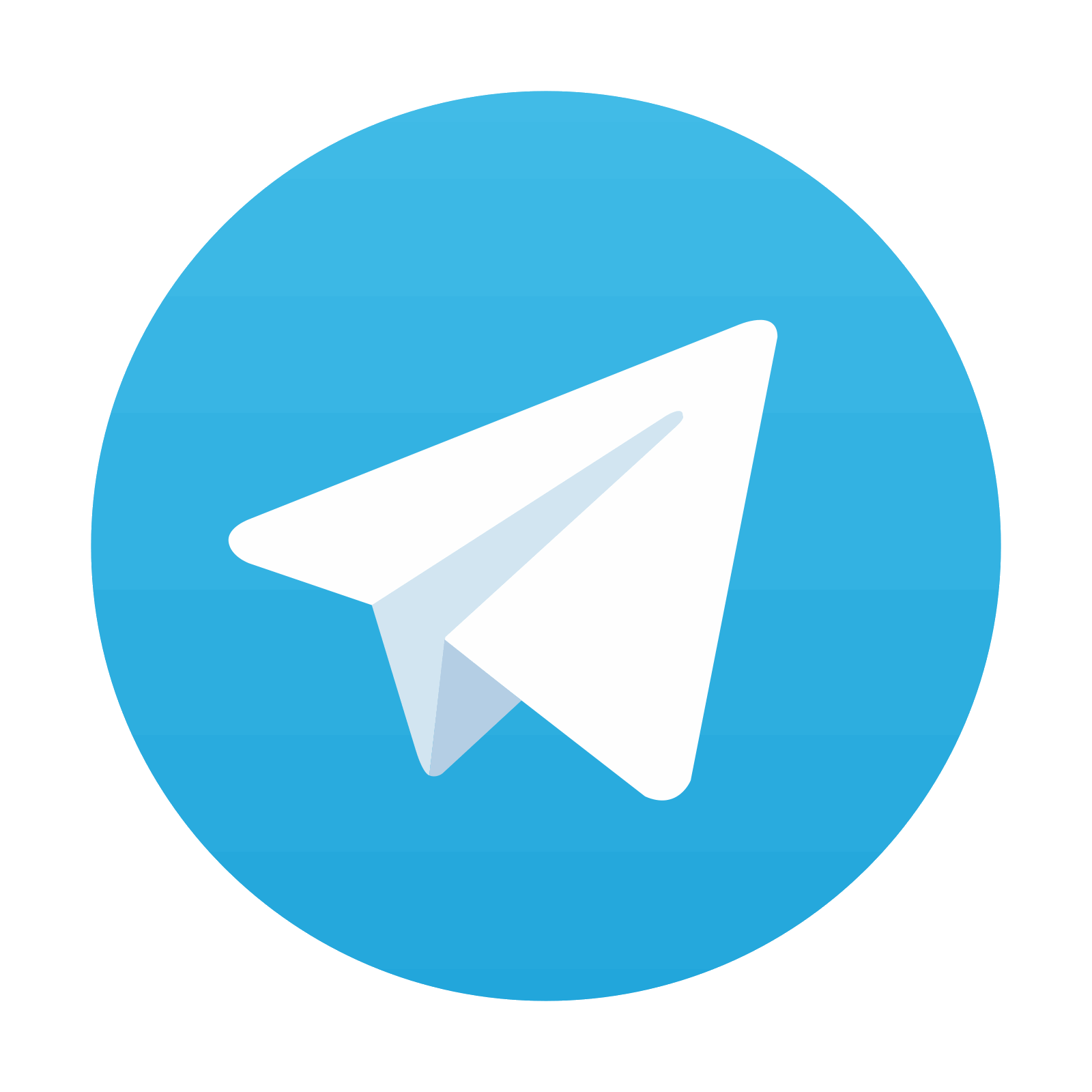
Stay updated, free articles. Join our Telegram channel
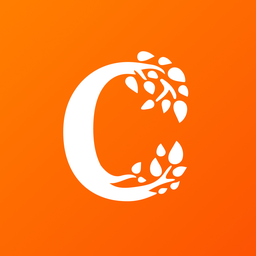
Full access? Get Clinical Tree
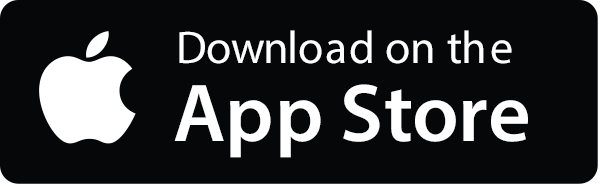
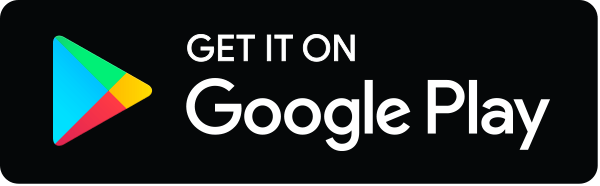