Abstract
Erythropoietin (EPO) was first identified as a regulator of erythropoiesis. Additional studies have demonstrated a myriad of pleotropic effects (eg, an ability to decrease inflammation, oxidative stress, and apoptosis). Further, EPO facilitates both angiogenesis and neurogenesis and modulates core components of primary and secondary brain injury. Animal models have confirmed the therapeutic potential of EPO in both experimental ischemic and traumatic brain injury (TBI). Unfortunately, clinical studies in stroke, TBI, and other related neurological injuries/disorders have thus far failed to demonstrate meaningful improvements after interventions with EPO. The surprising failure of EPO in clinical trials may be linked to inadequate dosing regimens, incorrect timing of treatments, duration of the therapy, and/or to inability (eg, lack of sensitivity) to detect outcomes reflective of clinical improvement. As such, optimized administration of EPO or EPO derivatives may provide a novel therapeutic approach for treatment of currently intractable brain injuries and illnesses.
Keywords
Angiogenesis, Cerebrovascular reactivity, Erythropoietin, Neurogenesis, Progenitor cells
Acknowledgments
This work was supported by Henry Jackson Foundation Award Number: 306135-7.01-60855 (USU Site No. G192JI), by the Center for Neuroscience and Regenerative Medicine at the Uniformed Services University of the Health Sciences, and by the National Institutes of Health, National Institute of Neurological Disorders and Stroke, Intramural Research Program.
Neurodegeneration and Neurogenesis After Traumatic Brain Injury
The pathophysiologic damage that results from traumatic brain injury (TBI) is shaped by both primary and secondary mechanisms of injury. Primary injury is a consequence of both the initial mechanical impact sustained by brain tissue and/or the inertial forces that cause cellular strain and membrane damage ( ). Secondary injury includes ionic imbalance, the release of excitatory amino acids, mitochondrial dysfunction/oxidative damage, inflammation, apoptosis, and diffuse axonal injury ( ). A compromised blood–brain barrier (BBB) contributes to the propagation of vasogenic and cytotoxic edema and allows the infiltration of inflammatory cytokines and chemokines into the brain parenchyma, ultimately promoting the infiltration of inflammatory cells ( ) ( Fig. 4.1A and B ). The secondary injury cascade ultimately results in the activation of proteases (eg, calpains and caspases), which contribute to cell death via either apoptosis or necrosis ( ). The mechanisms of secondary injury develop within hours to days after the primary injury and thereby provide a realistic window for clinically relevant therapeutic interventions.

Cellular damage resulting from secondary injury mechanisms in TBI are followed by a restorative phase during which the brain attempts to remodel itself in an effort to compensate for damage. Posttraumatic plasticity has been shown to involve neurogenesis, gliogenensis, angiogenesis, synaptic plasticity, and axonal sprouting ( ). Accordingly, compensatory plasticity is believed to underlie the spontaneous recovery of function that takes place after TBI, with its ultimate extent depending on severity of TBI, age, and other factors ( ). It is prudent to note that the processes underlying plasticity can be augmented by growth factors (and other growth related proteins) and thus may be modified/improved via pharmacologic interventions ( ).
Of prime importance is the widely accepted view that a decrease in cerebral oxygenation is an important pathologic component of secondary brain injury. A study of severe TBI patients showed that a hemoglobin (Hb) level lower than <9 g/dL was associated with decreased brain tissue oxygenation (PbtO 2 ), while an anemic status (ie, Hb <9 g/dL) and a simultaneously low PbtO 2 (ie, <20 mmHg) increased the risk of unfavorable clinical outcomes ( ). In line with the aforementioned, a study has shown that patients with severe TBI and low Hb levels (10.7 ± 0.9 g/dL) had lower survival rate than those with higher Hb levels (12.9 ± 0.4 g/dL) ( ). As such, a number of therapies have demonstrated preclinical efficacy via a modulation of tissue oxygenation and have thus improved neurologic function(s) in animal models of TBI ( ). Of these, the agent with the most comprehensive and convincing preclinical data (suggestive of clinical efficacy) in TBI is erythropoietin (EPO) ( ).
Erythropoietin as an Endogenous Neuroprotective Molecule
The EPO gene is located on chromosome 7 and encodes for a ∼30-kDa glycoprotein, which is expressed mainly in the peritubular fibroblasts of the kidney and in liver hepatocytes ( ). When tissue becomes hypoxic, hypoxia-inducible factor-1α (HIF-1α) is stabilized and activates the EPO gene ( ). EPO production and the resultant increase in erythropoiesis leads to improved tissue oxygenation and a subsequent downregulation of HIF ( ). In addition to the kidneys, EPO has been shown to be produced within the brain by both astrocytes and neurons ( ). It is therefore unsurprising that the EPO system plays an important role during normal central nervous system (CNS) development ( ). Mice with targeted deletions of EPO display an increase in CNS apoptosis before the development of severe anemia, suggesting that the EPO required for normal brain development acts in a manner distinct from its role in erythropoiesis ( ).
EPO expression within the brain increases after pathologic insults and injuries. Most notably, local CNS EPO production is increased during hypoxia (eg, experimental anemia and hypoxia induced via carbon monoxide exposure resulted in 20-fold upregulation of EPO mRNA) ( ). Increased local production of endogenous CNS EPO suggests that EPO may act in a paracrine and/or autocrine fashion in an effort to facilitate endogenous neuroprotection.
Neuroprotective effects of EPO have been extensively demonstrated for a number of cell types within the brain. For example, EPO was able to protect hippocampal and cortical neurons from glutamate neurotoxicity and also reverse hypoxia-induced hippocampal neuronal death when applied in conjunction with the hypoxic insult ( ). EPO also aids the maturation of glia (ie, oligodendrocytes and astrocytes) ( ). Finally, EPO has been shown to maintain microglial integrity during oxidative stress induced by oxygen and glucose deprivation, thereby fostering tissue repair and reorganization ( ).
The majority of the neuroprotective and other effects ascribed to EPO have been linked to the binding of EPO to erythropoietin receptors (EPO-R) ( ). EPO-R is a type I cytokine receptor that possesses a single transmembrane domain which lacks an intrinsic tyrosine kinase domain ( ). Binding of EPO to the EPO-R changes the conformation of the receptor to a dimer thereby activating the Janus family tyrosine protein kinase-2 (Jak2) and downstream signal transduction pathways which include phosphatidylinositol-3 kinase (PI3K), mitogen-activated protein kinases (MAPK), and signal transducers and activators of transcription-5 (STAT5) ( ) ( Fig. 4.2 ).

EPO-R expression within the brain has been demonstrated during both development and adulthood in humans and other higher mammals ( ). The highest levels of EPO-R expression are detected in the neural tube of the mouse at embryonic day 10.5 and are comparable to levels found within adult hematopoietic tissue ( ). In the adult brain EPO-R are predominantly located within the midbrain, cortex, hippocampus, and capsula interna ( ). Within these niches, EPO-R are present on various cell types, such as neurons, astrocytes, oligodendrocytes, and brain endothelial cells ( ). Human, rat, and mouse brain capillaries and stellate astrocytes display an intense immunoreactivity for EPO-R, while large vessels and other astroglia do not express EPO-R ( ). Transmission electron microscopy has shown EPO-R on the surface of capillary endothelial cells and within the astrocytic endfeet surrounding them ( ). Interestingly, brain capillary endothelial cells express two forms of EPO-R mRNA, which are differentially activated after the administration of human recombinant EPO (rHu EPO) ( ). Such a multicellular distribution of EPO-R again suggests that EPO may function more generally (ie, beyond driving erythropoiesis) to aid in tissue development and repair.
Beyond normal development/homeostatic biology EPO-R expression is increased via a myriad of pathologic conditions. Mild hypoxia increases EPO-R expression in the ventral limbic region of the rat brain ( ), and both EPO and EPO-R are upregulated in the human brain after ischemic infarcts and/or hypoxic damage ( ). Increased expression of EPO-R has also been noted in astrocytes of the hippocampus in patients with sporadic Alzheimer’s disease (AD) and mild cognitive impairment. This suggests that the upregulation of EPO-R in the temporal, cortical, and hippocampal astrocytes may be an attempt at neuroprotection during the pathogenesis of sporadic AD ( ).
EPO is a large molecule (molecular weight of ∼30 kDa), yet its ability to cross an intact BBB has been confirmed ( ). Murine, human, and darbepoetin alfa (an analog of human EPO in clinical use) EPO are all capable of crossing the murine BBB at the same rate as albumin suggesting that these proteins cross via extracellular pathways. Accordingly, EPO concentration in the brain was shown to peak ∼3 h after intravenous injection ( ). Of note, endogenous EPO has been found in the cerebrospinal fluid of patients with TBI at levels that correlated with the overall degree of BBB dysfunction ( ).
In summary, numerous animal studies have confirmed EPO’s neuroprotective, antiapoptotic, neurogenic, and angiogenic properties following both TBI and ischemic stroke ( ). Further, studies have demonstrated EPO’s ability to decrease microglial activation/activity after trauma possibly via the attenuation of secondary mechanisms of brain injury ( ). As such, preclinical studies in TBI, stroke, and other neurological disorders support the hypothesis that EPO may be therapeutic for both ischemic and traumatic brain injuries in humans.
Derivatives of Erythropoietin
One of the principal concerns underlying EPO therapy in human patients is that the administration of several doses may ultimately lead to potentially harmful increases of Hb with a concordant increase in the incidence of thrombotic sequela ( ). Therefore, a number of EPO derivatives which lack hematopoietic effects have been generated and studied both in vitro and in vivo in an effort to clarify their therapeutic potential ( ).
The most studied derivative thus far is carbamylated erythropoietin (CEPO). Carbamylation of the lysines within EPO interferes with its binding to the EPO-R on hematopoietic cells and thus prevents increases in hematopoietic activity after long-term administration ( ). The precise molecular mechanisms governing the actions of CEPO remain to be elucidated; because CEPO is not able to bind to classical EPO-R it may act by engaging an alternative receptor ( ). Critically, peripherally administered CEPO is able to cross the BBB and has been shown to be within the brain parenchyma and CSF compartments after middle cerebral artery occlusion (MCAO) ( ). A number of experimental studies in both stroke and TBI have demonstrated the neuroprotective effects of CEPO, which are comparable to effects produced by wild-type EPO ( ). For example, CEPO is capable of reducing CA1 pyramidal cell death in the hippocampus after TBI in a manner similar to EPO ( ). Further, postischemic treatments with CEPO reduced infarct size and concordantly improved neurological outcomes after focal cerebral ischemia in a manner analogous to EPO ( ). Several mechanisms associated with CEPO-mediated neuroprotection have been observed. First, similar to EPO, CEPO has antiapoptotic properties with regard to on-damaged neuronal cells ( ). Second, both EPO and CEPO promote angiogenesis via endothelial progenitor cells (EPC), which are derived from hematopoietic CD34 + progenitors ( ). EPC levels have been associated with stroke severity ( ) ( Fig. 4.3 ), and increases in EPC levels have been linked with functional recovery following ischemic stroke ( ). Studies on human EPC and erythroid cell lines have shown that CEPO prevents EPC death via a reduction in apoptosis ( ). Third, CEPO significantly increases neural progenitor cell differentiation into neurons ( ). Fourth, CEPO has been shown capable of reducing neuroinflammation (as measured by microglia activation and attenuation of polymorphonuclear leukocyte infiltration) after experimental stroke ( ). Despite such promising results in preclinical studies, human studies using CEPO in both stroke (NCT00756249) and Friedreich’s Ataxia (NCT01016366) have not reported any clinical benefits yet have reinforced the drug’s overall safety profile.

Another EPO analog, asialoEPO, was developed under the premise that a continuous presence of EPO is required for the production of red blood cells while only a brief exposure is necessary to induce neuroprotection. Accordingly, intravenously injected asialoEPO exhibits a plasma half-life of 1.4 min and is below the lower limit of detection within the systemic circulation at 1–2 h. This is in stark contrast to rhEPO, which has a plasma half-life of ∼5.6 h; in line with such a hypothesis multiple doses of asialoEPO do not increase hematocrit (Ht) levels ( ). Interestingly, the binding affinity of asialoEPO to an EPO-R-Fc construct falls within a similar range to that of rhEPO, thereby suggesting that asialoEPO is similar to EPO in its mechanism of action ( ); this differs strikingly from aforementioned CEPO ( ). AsialoEPO is able to cross the BBB and has been detected in the CSF of rats having undergone MCAO when it was administered for 4 days through the pump at a dose of 20 μg/kg per 24 h ( ). In studies on experimental stroke and models of traumatic spinal injury, asialoEPO acted as a nonerythropoietic cytokine with a broad range of neuroprotective activities ( ). Neuroprotection by asialoEPO was confirmed via a reduction of infarct size in an MCAO model when administered 90 min after the occlusion; further the administration of asialoEPO also diminished the zone of experimental spinal cord injury ( ). Another putative mechanism of action by asialoEPO (as shown in a model of a spinal cord injury) includes the promotion of glial activation ( ). Finally, both the safety and putative efficacy of asialoEPO were further demonstrated in a model of amyotrophic lateral sclerosis ( ).
A new nonhematopoietic EPO-R agonist ARA290 has displayed promising cytoprotective capacities and efficacy in both preclinical and clinical studies of metabolic neuropathy ( ). Currently administration of ARA290 shows potential in cardiac disorders, in neuropathic pain, and in sarcoidosis-induced chronic neuropathic pain ( ), although, studies focusing on CNS pathology have yet to be published.
Preclinical Studies of Erythropoietin in Stroke
The neuroprotective effects of EPO have been extensively studied in a wide range of stroke models. EPO markedly reduced astrocyte activation and the recruitment of leukocytes/microglia produced by MCAO thereby selectively attenuating cytokine production and neuroinflammation ( ). EPO was effective in a neonatal model of ischemia reducing death of endothelial cells (EC) within the ischemic penumbra, and increasing both EC and neuronal proliferation that supports the hypothesis that EPO is capable of promoting neurovascular repair ( ). Further studies using focal ischemia demonstrated that rhEPO treatment reduced brain edema after ischemia ( ). Expression of the BBB integrity markers, occludin, α-catenin, and β-catenin, in the brain were also preserved after administration of rhEPO ( ). A meta-analysis of EPO studies confirmed the preclinical efficacy of EPO in stroke ( ), yet acknowledged that further studies are needed to evaluate the effects of EPO in conjunction with other therapies (eg, thrombolysis) and with common human comorbidities ( ).
Erythropoietin in Other Animal Models of Brain Disease
The influence of EPO has been examined in various animal models of an assortment of brain pathologies (eg, multiple sclerosis, Parkinson’s disease, and epilepsy). Briefly, EPO has a protective role in experimental autoimmune encephalomyelitis (EAE), which is an animal model of multiple sclerosis. EPO reduced disease severity in myelin oligodendrocyte glycoprotein (MOG)-EAE mice and enhanced expression of an antioxidative protein heme oxygenase-1 (HMOX-1). Further, administration of EPO was associated with modulation of adaptive immunity, which manifested as a lower expression of interferon-γ, interleukin (IL)-23, IL-6, and IL-17 mRNA, and a significantly higher expression of IL-4 and IL-10 mRNA within the CNS of EPO-treated MOG-EAE mice versus controls ( ). EPO delayed seizures in the kainite model of status epilepticus when rhEPO in dose 5000 IU/kg administered 24 h before kainite-induced status epilepticus to mice delayed seizures, markedly reduced motor involvement, and reduced mortality ( ). In these models of brain disease EPO also exhibits pleotropic effects. For example, it influenced locomotor activity, the survival of nigral dopaminergic neurons, and nitrate levels within the substantia nigra and striatum in a 1-methyl-4-phenyl-1,2,3,6-tetrahydropyridine (MPTP)-induced animal model of Parkinson’s ( ). Furthermore, in the MPTP-induced animal model of Parkinson’s disease, EPO administration influenced antioxidant enzyme activities within the brain and decreased glutathione peroxidase activity in both the substantia nigra and striatum ( ).
Preclinical Studies of Erythropoietin in Traumatic Brain Injury
It is critical to note that there is not one animal model for TBI that is capable of recapitulating the multiple mechanisms of pathogenesis triggered by the diverse series of molecular and cellular events ( ) triggered by such an injury. Despite this limitation multiple experimental models of TBI have demonstrated the neuroprotective effects of EPO. In a model of frontal cortical TBI, the injection of EPO (5000 U/kg) resulted in a significant decrease in the volume of cavital injury at 10 days postinjury ( ). In a closed head model of TBI, the administration of 5000 U/kg of EPO at 1 and 24 h postinjury facilitated both motor and cognitive recovery, via a decrease in tissue inflammation, axonal degeneration, and brain tissue apoptosis ( ). Likewise, EPO was shown to be effective when administered 1 day after injury for 14 days at a dose of 5000 U/kg in a controlled cortical impact (CCI) model of TBI. In this model EPO increased the numbers of newly formed neurons identified via a double labeling method using bromodeoxyuridine and microtubule-associated protein-2. Additionally, the study showed that spatial memory markedly improved in the EPO group compared to controls ( ). These findings were supported by another work when EPO administered in dose 5000 U/kg at 6 h and 3/7 days after injury in CCI model significantly reduced lesion volume and cell loss in dentate gyrus, at the same time improving behavioral scores of sensomotor and spatial learning ( ).
It has been suggested that the neurorestorative effects of EPO may be explained by a concomitant increase in Ht levels. However, there was no relationship between neuroprotection and Ht levels in a CCI model when administration of EPO significantly reduced hippocampal cell loss, enhanced angiogenesis and neurogenesis with significant improvement of sensomotor outcomes ( ). Importantly, that normovolemic hemodilution after EPO treatment conducted in this study normalized Ht to the pretreatment level, but it did not affect the beneficial histological and functional outcomes of EPO therapy for TBI ( ). Finally, a recent meta-analysis confirmed the beneficial effects of EPO in experimental animal models of TBI. Of a total of 290 studies it was shown that statistically significant findings favor the beneficial effects of EPO after TBI in terms of both reductions in lesion volume and improvements in neurobehavioral outcomes ( ).
Erythropoietin Promotes Angiogenesis
As previously described, EPO is a potent physiologic stimulus for progenitor cells within the bone marrow and peripheral blood ( ). EPO promotes the formation of new vessels in part via an increase in the levels of circulating EPC ( ). EPO displays significant angiogenic potential, as it was able to induce capillary outgrowth after stimulation in a manner that was analogous to vascular endothelial growth factor (VEGF) ( ). Accordingly, weekly administration of EPO to anemic patients and to controls has resulted in increased EPCs by 300% as compared to the pretreatment levels ( ). Furthermore, a clinical study showed that 5000 IU of EPO when administered subcutaneously at 48 and 72 h after acute stroke increased EPC levels and promoted functional improvements ( ).
Human Trials With Erythropoietin During the Acute Phase of Ischemic Stroke and Traumatic Brain Injury
The safety and efficacy of EPO in critically ill patients has been studied in two large (ie, >2700 patients) multicenter randomized clinical trials (RCTs) ( ). These studies concluded that EPO significantly reduced mortality in trauma patients [hazard ratio (HR) 0.37, 95% confidence interval (CI) 0.19–0.72] ( ) but not in medical or nontrauma surgical patients.
EPO has also been studied as a treatment for ischemic stroke. In a Phase II clinical trial ( ), the intravenous administration of EPO was initiated within 5 h of stroke onset, and continued for 3 days, and resulted in improved neurologic outcomes 30 days after stroke as measured by the NIH Stroke Scale (NIHSS), a reduced infarct size as measured by magnetic resonance imaging (MRI) [diffusion-weighted imaging (DWI) and fluid-attenuated inversion recovery (FLAIR)], and reduced serum S100 calcium-binding protein β (S100β) concentrations. These investigators completed a Phase III study, which reported a higher mortality for those within the EPO-treatment group ( ). This finding was attributed to the higher rate of thrombolytic treatment with recombinant tissue plasminogen activator (in the enrolled acute stroke patients) and thus, has limited relevance when expanded to TBI populations (this treatment has no indications in TBI).
Due to the extensive preclinical data that indicates the potential efficacy of EPO as both a neuroprotective and neurorestorative agent, several RCTs have been conducted in TBI. The randomized study enrolled 200 patients with severe TBI, initiating EPO therapy within 6 h of injury. The study showed that neither the administration of EPO nor the maintenance of Hb concentrations greater than 10 g/dL resulted in improved neurological outcome at 6 months with the Glasgow Outcome Scale-Extended (GOS-E) score as the primary end point. Additionally, the transfusion threshold required to maintain Hb concentration of 10 g/dL was associated with a higher incidence of adverse events (AE) ( ). The Australia–New Zealand Intensive Care Research Centre conducted a multicenter study that enrolled 606 patients with moderate-to-severe TBI (GCS 3–12). The trial showed that EPO failed to reduce the number of patients with severe neurological dysfunction (GOS-E level 1–4) when the outcome was evaluated 6 months after the injury ( ). Of note, a small randomized trial on administration of EPO to patients with traumatic spinal cord injury compared rhEPO to administration of methylprednisolone. Three of the 11 patients who received EPO showed an improvement, reaching the primary end point of the study ( ). Table 4.1 summarizes the doses of EPO, reported outcomes, AE, and serious adverse events (SAE) of major clinical trials on EPO for adult populations.
Summary of EPO Dosing in Acute Clinical Trials | |||||||
---|---|---|---|---|---|---|---|
References | Patient Population/Trial Design | n | Dose | Frequency | Total Dose | Outcome | Serious Adverse Events/Adverse Events (SAEs/AEs) |
| Critical illness. Phase III RCT | 1302 | 40,000 U starting day 3 | 40,000 U weekly × 3 | 120,000 U over 2 weeks | Decreased need for RBC transfusion in EPO group | No significant difference between placebo and EPO. Trend toward benefit in EPO group having fewer CNS SAEs ( p = .08) |
| Critical illness. Phase III RCT | 1460 | 40,000 U starting day 3 | 40,000 U weekly × 3 doses | 120,000 U over 2 weeks | Decreased need for RBC transfusion in EPO group | Decreased mortality in EPO group. Increased clinically relevant thrombotic event in EPO group ( p = .008) |
| Stroke. Phase II RCT | 53 | 33,000 U starting hour 8 | 33,000 U daily × 3 doses | 100,000 U over 48 h | Improved NIHSS, Rankin SS, and Barthel Index in EPO group | No differences in AEs or SAEs |
| Stroke. Phase III RCT | 522 | 40,000 U starting hour 6 | 40,000 U daily × 3 doses | 120,000 U over 48 h | No difference NIHSS, RSS, BI | Increased death in EPO group (OR 1.98, p = .01) |
| Stroke. Reanalysis of Phase III RCT | 163 (no tPA) | 40,000 U starting hour 6 | 40,000 U daily × 3 doses | 120,000 U over 48 h | Lower brain injury biomarkers in EPO group | |
| CAD, before CABG. Phase II RCT | 182 | 150 U/kg or 300 U/kg, before CABG | 3 doses (−5, 0, 2 days from CABG) | Calculated for 60 kg : 27,000 U or 54,000 U | No benefit reducing transfusion requirements | No difference in AEs or SAEs |
| CAD, after PCI reperfusion. Phase II RCT | 138 | 33,000 U starting after PCI | 33,000 U × 3 doses at 0, 24, 48 h | 100,000 U over 48 h | No benefit on infarct size, LVEF | Trend to increased recurrent vascular event ( p = .15) |
| CAD, after PCI reperfusion. Phase III RCT | 529 | 60,000 U starting after PCI | Single dose | 60,000 U | Decreased recurrent cardiovascular events ( p = .03) | Fewer cardiac SAEs in EPO group |
| CAD, after PCI reperfusion. Phase II RCT | 110 | 1000 U/kg after PCI | Single dose | Calculated for 60 kg : 60,000 U | EPO decreased microvascular occlusion. No difference infarct size or left ventricle function at 3 months | No difference in AEs or SAEs |
| Severe TBI. Phase II RCT | 16 | 40,000 U starting hour 6 | Single dose | 40,000 U | No difference in brain injury biomarkers | No difference in AEs or SAEs |
| Severe TBI. Retrospective matched case–control | 267 | Dose not provided in paper: 89 participants treated with EPO, c/w 178 who were not | Decreased mortality in EPO group | No significant differences in SAEs/AEs | ||
| Moderate and severe TBI. Phase III RCT | 606 | 40,000 U starting 24 h after TBI | 40,000 U weekly × 3 | 120,000 U over 2 weeks | No reduction in the number of patients with severe neurological dysfunction (GOS-E levels 1–4) | No increase of deep vein thrombosis in EPO group |
| Bipolar disorder and treatment-resistant depression | 80 (target) | 40,000 IU, IV | Once weekly for 8 weeks | 320,000 IU over 8 weeks | EPO enhanced sustained attention ( p = .001), recognition of happy faces ( p = .03), and speed of complex information processing ( p = .01) ( ) and reduced depression composite at weeks 9 and 14 ( p values = .02) ( ) | No difference in SAE |
| Schizophrenia Phase II RCT | 32 | 40,000 IU, IV | Once weekly for 3 months | 480,000 IU over 12 weeks | Decrease of brain atrophy | No significant differences in SAEs/AEs |
| Schizophrenia Phase II RCT | 39 | 40,000 IU, IV | Once weekly for 3 months | 480,000 IU for 12 weeks | Improvement in schizophrenia-related cognitive performance | No significant differences in SAEs/AEs |
| Severe TBI enrolled within 6 h of injury | 102 | 500 IU/kg per dose IV | Daily for 3 days and then weekly for 2 more weeks | Up to 200,000 IU over 2 weeks | Glasgow Outcome Scale score dichotomized at 6 months postinjury | Increased incidence of upper extremity DVT in the EPO 2 regimen group ( p = .003) |
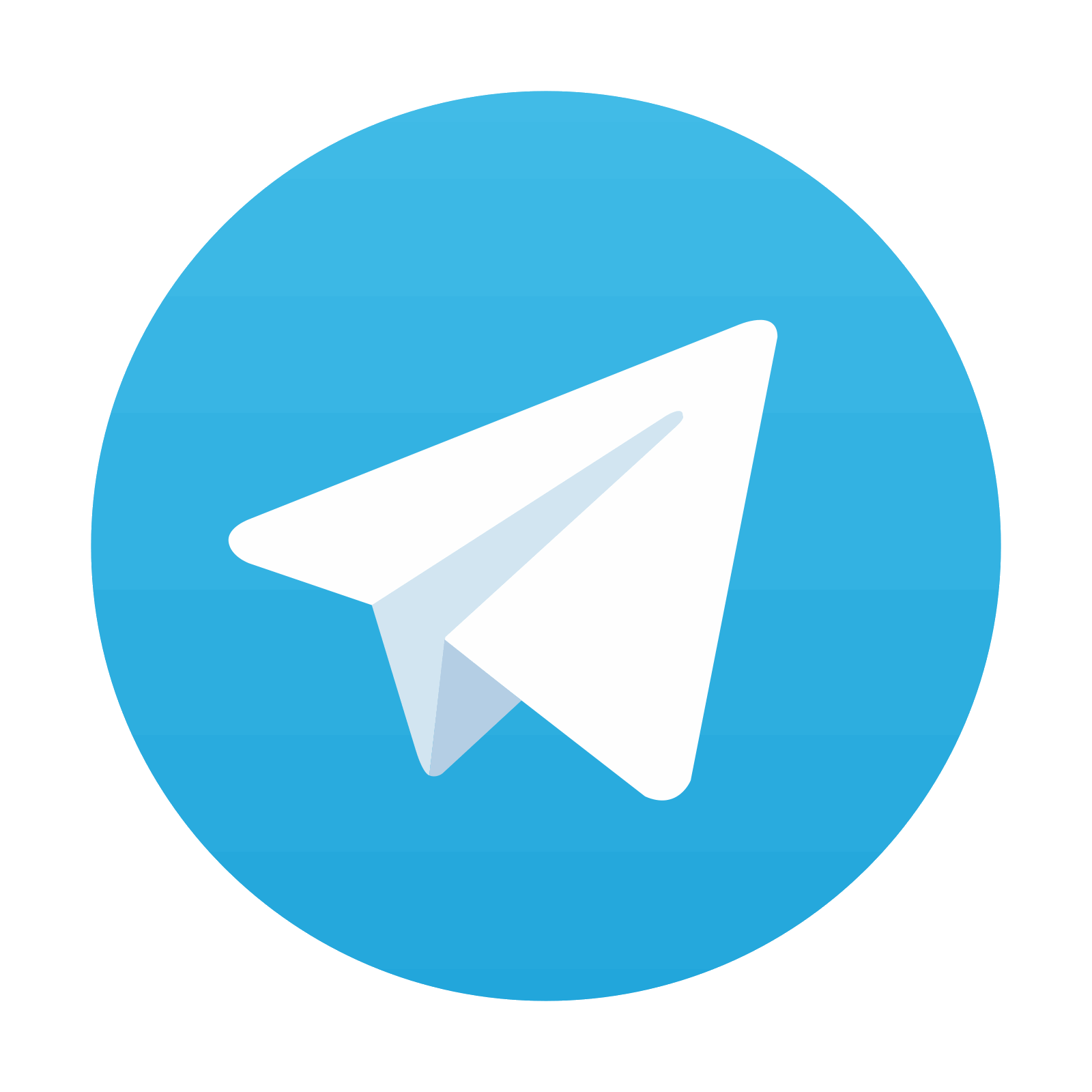
Stay updated, free articles. Join our Telegram channel
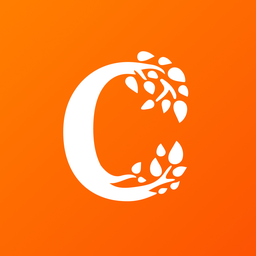
Full access? Get Clinical Tree
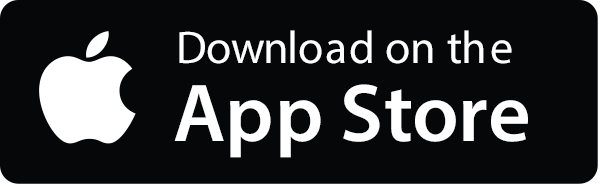
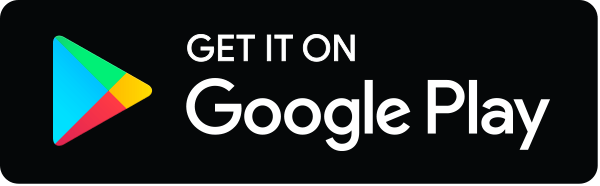
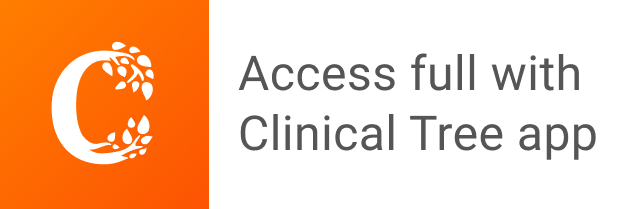