The major excitatory neurotransmitter in the brain is glutamate; the major inhibitory neurotransmitter is γ-aminobutyric acid (GABA).
Glutamate receptors comprise two large families, ligand-gated ion channels called ionotropic receptors and G protein–coupled receptors called metabotropic receptors.
Ionotropic glutamate receptors are divided into three classes—α-amino-3-hydroxy-5-methyl-4-isoxazole propionic acid (AMPA) receptors, kainate receptors, and N-methyl-D-aspartate (NMDA) receptors—which are named after synthetic ligands that activate them. Individual excitatory synapses typically express several different subtypes of ionotropic glutamate receptors as well as metabotropic receptors.
In contrast to AMPA receptors and kainate receptors, the NMDA receptor has two important biophysical properties. Because it is highly permeable to calcium and is voltage dependent, it allows calcium entry only if the cell is depolarized.
AMPA receptors mediate the vast majority of excitatory synaptic transmission in the brain, whereas NMDA receptors play an important role in triggering synaptic plasticity and, when overactivated, in triggering excitotoxicity.
There are eight subtypes of metabotropic glutamate receptors. When localized to the presynaptic terminal, they inhibit neurotransmitter release. When localized to the postsynaptic membrane, they exert complex modulatory effects through specific signal transduction cascades, which can lead to excitatory or inhibitory effects.
The most extensively studied form of synaptic plasticity is long-term potentiation (LTP) in the hippocampus, which is triggered by strong activation of NMDA receptors and the consequent large rise in postsynaptic calcium concentration. Several other types of LTP are widespread in the nervous system and are mediated via distinct mechanisms.
Long-term depression (LTD), a long-lasting decrease in synaptic strength, also occurs at most excitatory and some inhibitory synapses in the brain.
The GABAA receptor, a ligand-gated chloride channel, and the GABAB receptor, a G protein–coupled receptor, are the two major classes of GABA receptors.
GABAA receptors, which are highly heterogeneous, mediate the bulk of inhibitory synaptic transmission in the brain. Many drugs, most notably benzodiazepines and barbiturates, bind to GABAA receptors and enhance their function.
GABAB receptors are localized both presynaptically, where they inhibit neurotransmitter release, and postsynaptically, where they mediate a slow, inhibitory synaptic response.
Glycine, like GABA, is an inhibitory neurotransmitter that activates receptors that are ligand-gated chloride channels. It is critical in inhibitory neurotransmission in the spinal cord and brainstem.
Amino acids are the building blocks of proteins involved in normal intermediary metabolism, but they can also function as neurotransmitters. The amino acids glutamate and, to a much lesser extent, aspartate mediate most of the fast excitatory synaptic transmission in the brain; likewise, the amino acids γ-aminobutyric acid (GABA) and, to a lesser extent, glycine mediate most fast inhibitory synaptic transmission. Excitatory amino acids are utilized by nearly every information-bearing circuit in the brain and have been implicated in such diverse pathologic processes as epilepsy, ischemic brain damage, and several psychiatric disorders. In addition, they are necessary for the development of normal synaptic connections. Consequently, amino acid neurotransmitters have been the subject of intensive research for many decades.
Long before glutamate’s role in neurotransmission was established, investigators observed that glutamate excites most neurons in the CNS. In fact, it is responsible for most neurotransmitter action at excitatory amino acid receptors. Glutamate is present in high concentrations in the adult CNS and is released in a Ca2+-dependent manner by electrical stimulation. Enzymes responsible for glutamate synthesis and degradation are located in both neurons and glial cells, as are high-affinity glutamate receptors and excitatory amino acid reuptake transporters (EAATs), which terminate the synaptic actions of glutamate. Many of these reuptake and receptor proteins also respond to aspartate, which may mediate transmission at a small number of central excitatory synapses.
Glutamate and aspartate are charged amino acids and therefore do not cross the blood–brain barrier. As a result, they must be synthesized in the brain from glucose and a variety of other precursors. Glutamate, which is the reduced form of glutamic acid, is in a metabolic pool with α-oxoglutaric acid and glutamine. It is packaged into presynaptic vesicles via several steps 5–1. After action potential–driven release, glutamate is primarily taken up by glial cells, where it is converted into glutamine by glutamine synthetase. The resulting glutamine is transported out of glia by system N-1 (SN1), a Na+– and H+-dependent pump that is homologous to the vesicular GABA transporter (VGAT). Glutamine is subsequently taken up by neurons, by means of a transport process that remains poorly described, and is converted back into glutamate by glutaminase. As discussed later in this chapter, glutamine also replenishes the transmitter pool of GABA.
5–1
Synthesis and degradation of the neurotransmitter pool of glutamate. In the mitochondrial compartment of glutamatergic nerve terminals, glutamine (gln) is converted to glutamate (glut) by the enzyme glutaminase. Alternatively, glutamate is synthesized by the transamination of aspartate (asp) by transaminase. Newly synthesized glutamate is packaged into synaptic vesicles by means of a Mg2+– and ATP-dependent proton gradient–coupled uptake process. After it is released from nerve terminals, most of it is taken up into glial cells, where it is converted into glutamine by glutamine synthetase. Glutamine is then pumped out of the glia by SN1 and taken up by nerve terminals, where it is converted back to glutamate to replenish the transmitter pool. Uptake of glutamate into glial (and, to a lesser extent, neural) compartments is achieved by the Na+-dependent glutamate transporters EAAT1 to 3.

Synaptic vesicles actively accumulate glutamate through a Mg2+– and adenosine triphosphate (ATP)-dependent uptake process that is driven by an electrical gradient across their membranes. During uptake, concentrations of glutamate in these vesicles likely exceed 20 mM. Substances that destroy the electrochemical gradient across vesicular membranes prevent the transporters from concentrating this amino acid. The three isoforms of the vesicular glutamate transporter, VGluT1 to 3, are highly selective with a high affinity for glutamate and a low affinity for aspartate (Chapter 3).
After release from presynaptic terminals, the reuptake of glutamate and aspartate serves to control the extracellular concentrations of these amino acids in the CNS. Na+-dependent glutamate plasma membrane transporters are coupled to electrochemical gradients for Na+, K+, and H+ 5–1. Because such coupling permits the transport of glutamate and aspartate against their concentration gradients, glutamate transporters are capable of decreasing extracellular glutamate concentrations to submicromolar levels. These transporters take up glutamate and aspartate with similar affinity and maximum velocity (Vmax). Glutamate transport is electrogenic, resulting in the net inward movement of positive charge during each transport cycle.
Five principal members of the high-affinity Na+-dependent family of glutamate plasma membrane transporters have been cloned: EAAT1 (also known as glutamate–aspartate transporter [GLAST]), EAAT2 (glutamate transporter-1 [GLT-1]), EAAT3 (excitatory amino acid carrier-1 [EAAC1]), and EAAT4 and 5. Each of these transporters belongs to a large superfamily whose members transport a wide range of neurotransmitters and related substances (Chapter 3). EAAT1 and 2, which are expressed by glial cells, appear to be responsible for the majority of glutamate reuptake in the CNS (see 5–1).
Several pathologic conditions, such as ischemia, can lead to the accumulation of glutamate or aspartate in extracellular spaces, which results in the excessive activation of glutamate receptors (Chapters 18 and 20). Such activation can in turn cause a variety of pathologic changes and can, in its extreme form, result in cell death. Glutamate transporters limit the concentrations of free glutamate and aspartate in extracellular spaces, and thereby prevent the excessive stimulation of glutamate receptors. Consequently, agents capable of facilitating transporter function might limit the damage caused by ischemia and other neurologic insults that cause extracellular glutamate to increase. However, most progress to date has involved developing inhibitors of glutamate transporters. Two such inhibitors are D,L-threo-3-hydroxyaspartate (THA), a broad-spectrum antagonist of glutamate and aspartate transport, and dihydrokainate (DHK), an inhibitor selective for the glial EAAT2 transporter. Although these drugs are useful experimental tools, they have no apparent clinical use.
Glutamate receptors comprise two large families: the ionotropic and the metabotropic receptors. Ionotropic glutamate receptors contain associated ion channels that are gated by agonist binding. Three classes of ionotropic glutamate receptors, N-methyl-D-aspartate (NMDA), α-amino-3-hydroxy-5-methyl-4-isoxazole propionic acid (AMPA), and kainate receptors, were originally named based on the ability of these drugs to serve as selective agonists 5–1.
Functional Classes | Gene Families | Agonists | Antagonists |
---|---|---|---|
Ionotropic | |||
AMPA |
|
|
|
Kainate |
|
|
|
NMDA |
|
|
|
Metabotropic | |||
Group I |
|
|
|
Group II |
|
|
|
Group III |
|
|
|
Metabotropic glutamate receptors (mGluRs) belong to the large superfamily of G protein–coupled receptors. These receptors, which are characterized by seven transmembrane domains (TMs), couple to G proteins and in turn mediate the biologic effects of receptor activation (Chapter 4). The term metabotropic was used to indicate that these receptors affect cellular biochemical (“metabolic”) processes, and do not form ion channels. However, mGluRs, like other G protein–coupled receptors, can exert profound effects on neuronal function through the regulation of other ion channels, second messenger cascades, and protein phosphorylation.
Synaptically released glutamate interacts with postsynaptic receptors located on dendrites or nerve terminals of immediately adjacent cells or on the nerve terminals from which glutamate is released. The binding of glutamate to AMPA and NMDA receptors opens postsynaptic cation channels and initiates a two-component excitatory postsynaptic current (EPSC) at most central synapses 5–2. Considerable evidence suggests that AMPA and NMDA receptors colocalize at most functional excitatory synapses. However, the ratio of AMPA to NMDA receptors at individual synapses can vary greatly; indeed, some synapses may contain only NMDA or AMPA receptors. In contrast, smaller numbers of kainate receptors are present in most CNS regions.
5–2
Synaptically released glutamate interacts with postsynaptic AMPA and NMDA receptors. A. Excitatory postsynaptic currents (EPSCs) caused by glutamate in a hippocampal neuron. When glutamate binds to NMDA and AMPA receptors, postsynaptic cation channels open, initiating a two-component EPSC. These components can be pharmacologically separated. Blockade of NMDA receptors by the antagonist D-APV (left) reveals a rapid AMPA receptor–mediated component of the EPSC. Conversely, blockade of AMPA receptors by CNQX (right) reveals a slowly rising and decaying NMDA receptor–mediated component of the EPSC at positive voltages. In contrast, application of D-APV at negative voltages (lower left) reveals little NMDA receptor contribution to the EPSC due to the profound block of these channels by Mg2+ ions. B. The current–voltage relationship for AMPA receptors. This relationship is roughly linear and reverses at approximately 0 mV, indicating that the channels do not discriminate well between Na+ and K+ ions. C. The current–voltage relationship for NMDA receptors. The concentration of Mg2+ in the extracellular fluid of the brain (approximately 1 mM) is sufficient to virtually abolish ion flux through NMDA receptor channels at membrane potentials close to resting potential (approximately −70 mV). As the membrane potential becomes less negative or even positive, the affinity of Mg2+ for its binding site decreases, permitting the passage of ionic current. Removal of extracellular Mg2+ linearizes the current–voltage relationship and permits significant current flow at negative voltages.

The activation of an AMPA receptor mediates a synaptic current that has a rapid onset and decay, whereas the current mediated by an NMDA receptor has a slower onset and a decay that lasts as long as several hundred milliseconds (see 5–2). The decay time of the NMDA receptor–mediated current is approximately 100 times longer than the mean open time of its channel. Such prolonged activation is believed to be caused by glutamate’s high affinity (Kd = 3–8 μM) for and consequent slow dissociation from these receptors. In contrast, glutamate has a much lower affinity for AMPA receptors (Kd = 200 μM), from which it rapidly dissociates.
AMPA receptors respond to single vesicles of glutamate. NMDA receptors, however, because of their special voltage dependence (described later in this chapter), commonly require coordinated input from many synapses for substantial activation. This allows NMDA receptors to act as coincidence detectors that can sense the activity of many independent synaptic inputs converging on the same cell 5–3.
5–3
The NMDA glutamate receptor as a coincidence detector. A. A single synaptic input results in the generation of a short-lasting excitatory postsynaptic potential (EPSP) that is mediated entirely by AMPA receptors. B. When multiple inputs occur simultaneously, the same single synaptic input generates a longer-lasting EPSP that is mediated by both AMPA and NMDA receptors. Thus, the NMDA receptor can “sense” the activity in adjacent inputs.

Current is carried through AMPA receptors primarily by the movement of Na+ from the extracellular space into the intracellular compartment. However, because the reversal potential of current (the membrane potential at which net current flow is zero; see Chapter 2) through AMPA channels is close to 0 mV, an outward current carried by K+ must counterbalance the inward flow of Na+ ions. The resulting current–voltage relationship for these AMPA receptors is roughly linear (see 5–2).
Some AMPA receptors, on both neurons and astrocytes, are also permeable to Ca2+. The translocation of Ca2+ from the extracellular space to the intracellular compartment plays a key role in the regulation of several second messenger systems. Thus, the permeability of some AMPA receptors to Ca2+ may have great functional importance, particularly in cells that do not contain NMDA receptors (which can always flux Ca2+). AMPA receptor channels that are permeable to Ca2+ lack the GluA2 subunit (see below) and exhibit an inwardly rectifying type of current–voltage relationship; they pass current readily in the inward direction but not in the outward direction. This occurs because intracellular polyamines such as spermine and spermidine, which associate with the channel, prohibit outward current from passing through. A spermine analogue, N(1)-acetylspermine (NASPM), a selective antagonist of GluA2-lacking AMPA receptors, is a valuable tool to study the influence of such receptors in brain function and plasticity.
All AMPA receptors can be blocked selectively by certain quinoxaline diones, the most notable of which is 6-nitro-7-sulfamobenzo-quinoxaline 2,3-dione (NBQX; see 5–1). NBQX is a potent competitive antagonist of AMPA receptors, which is reasonably selective for AMPA receptors over kainate receptors. Drugs in the 2,3-benzodiazepine class, such as GYKI 53655, are noncompetitive, selective antagonists of AMPA receptors and are being explored as neuroprotective drugs for the treatment of stroke. Because it has minimal effects on kainate or NMDA receptors, this class of drug permits unequivocal separation of the AMPA receptors from other categories of glutamate receptors.
AMPA and kainite receptors desensitize within milliseconds of exposure to agonist and can be reliably distinguished from one another by their response to two drugs, cyclothiazide and the lectin concanavalin A. Cyclothiazide relieves AMPA receptor desensitization but does not affect kainate receptors. In contrast, concanavalin A relieves the desensitization of kainate receptors, most likely by interacting with surface sugar chains, but does not have significant effects on AMPA receptors.
The possibility that enhancement of AMPA receptor function may be beneficial therapeutically has received increased attention with the development of positive allosteric modulators (PAMs) of AMPA receptors, also termed AMPAkines or AMPA potentiators. These drugs enhance AMPA receptor–mediated excitatory synaptic transmission by slowing deactivation or desensitization of the receptors, with the former mechanism appearing to be more functionally important. Clinical trials are currently being conducted in a wide range of neuropsychiatric disorders (eg, Alzheimer disease, depression, and attention deficit hyperactivity disorder [ADHD]) to determine whether this class of compound may be clinically useful.
Three families of ionotropic glutamate receptor subunits, encoded by at least 16 genes, assemble to form functional AMPA, NMDA, or kainate receptors (see 5–1). Within each family there is greater than 80% identity at the amino acid level over membrane-spanning domains. Between families, a lower degree of identity exists (~50%). Ionotropic glutamate receptors are believed to exist as tetramers. Different subunit combinations produce functionally different glutamate receptors. Moreover, there are striking regional differences in the expression of genes that encode these subunits.
Four subunits, termed GluA1 to GluA4 (also known as GluR1–GluR4), coassemble to form AMPA receptors. Each of these subunits is encoded by a distinct gene, and each exists in two forms, termed flip and flop. The flip and flop forms, which are generated by alternative splicing, exhibit region-specific patterns of expression in the brain, and give rise to receptors that differ in desensitization rates. The best characterized AMPA receptor subunit is GluA1, which is predicted to be 889 amino acids long; other ionotropic receptor subunits, such as those of nicotinic, GABAA, or glycine receptors, are approximately 420 amino acids long. The extra length of GluRs results from unusually large N-terminal extracellular domains.
AMPA and kainate glutamate receptor families have a topology that differs from that of certain other ligand-gated ion channels, such as GABAA and glycine receptors 5–4. Glutamate receptor subunits possess only three transmembrane-spanning domains. What appears to be an abridged TM, located between TM2 and TM3 in glutamate subunits, is a reentrant loop whose both ends face the cytoplasm. Homology mapping of glutamate receptors suggests that agonist binding requires portions of both the large N terminus and the short region between TM1 and TM2 (see 5–4).
5–4
Membrane topology of AMPA glutamate, GABAA, and glycine receptors. A. AMPA glutamate receptor subunits (GluA1–4) possess only three transmembrane-spanning domains. The channel-lining domain between TM1 and TM2 is a reentrant loop with both ends facing the cytoplasm. The Q/R site, which controls the Ca2+ permeability of AMPA receptor subunits, is located in this loop. The flip–flop site, located extracellularly between TM2 and TM3, yields two splice variants of each subunit. The glutamate-binding site of AMPA receptors is formed by several amino acids in the N-terminal and extracellular loop. B. In contrast to AMPA receptors, GABAA and glycine receptors possess four putative membrane-spanning domains.

As stated earlier, AMPA receptors lacking the GluA2 subunit are highly permeable to Ca2+ ions and are commonly found on GABAergic inhibitory interneurons throughout the brain. This Ca2+ permeability has been traced to a single amino acid in the reentrant loop of GluA2, which is known as the Q/R site (see 5–4). In GluA1, GluA3, and GluA4, a glutamine (Q) resides at this position, but in GluA2 an arginine (R) is present. When heteromeric receptors contain GluA2, they are relatively impermeable to Ca2+, most likely because the positive charge of the arginine residue repels Ca2+ from the channel pore. The replacement of glutamine with arginine in GluA2 occurs because of a process called RNA editing: the gene for GluA2 encodes a glutamine, and the unedited form of GluA2 is expressed in various regions of the brain during development. However, virtually all of the GluA2 mRNA present in adult mammalian brain is edited to a codon that encodes arginine at this site. This editing, which is catalyzed by adenosine deaminases acting on RNA (ADARs), changes a single base within GluA2 transcripts. In addition to Ca2+ permeability, the Q/R site of GluA2 influences the single channel conductance properties of associated AMPA receptors and the sensitivity of the receptor complex to blockage by polyamine spider toxins and polyamines (eg, NASPM). AMPA receptors that do not contain edited GluA2 show greater overall conductance. In addition, there is voltage-dependent inhibition of outward current flow by endogenous intracellular polyamines at positive membrane potentials giving rise to the inward rectification that is characteristic of GluA2-lacking AMPA receptors. A deficiency in GluA2 editing has been implicated in the pathophysiology of amyotrophic lateral sclerosis (ALS), acting perhaps by increasing Ca2+ flux into motor neurons and promoting excitotoxicity (Chapter 18).
The flip and flop splice variants of receptor subunits GluA1 to GluA4, together with the products of Q/R editing, yield a wide range of AMPA receptor subunit proteins. This diversity provides neurons with an extraordinary degree of flexibility in the construction of AMPA receptors. The degree of AMPA receptor heterogeneity employed by neurons in vivo remains unknown. Ca2+-permeable AMPA receptors lacking the GluA2 subunit are consistently found at excitatory synapses on GABAergic inhibitory interneurons, whereas most principal cells (ie, projection neurons) express Ca2+-impermeable AMPA receptors that contain the edited form of GluA2. There is growing evidence that the number of GluA2-lacking AMPA receptors at particular synapses is subject to dynamic regulation and may be an important mechanism of neural and behavioral plasticity.
Kainate receptors, like AMPA receptors, are cation-selective ligand-gated ion channels that strongly depolarize neurons when activated. They are found on presynaptic terminals, of both excitatory and inhibitory synapses, where their activation can modify neurotransmitter release, both because of their depolarizing actions and because, depending on their molecular composition, they are permeable to Ca2+. Depending on the synapse and the degree of activation, presynaptic kainate receptors can either facilitate or depress transmitter release. Kainate receptors are also found postsynaptically on certain neurons, where they normally generate slow, small, but functionally important, postsynaptic potentials. In the hippocampus and cortex, they may be particularly important in the early development of neural circuits. Recent data suggest that kainate receptors can also be metabotropic, initiating a G protein signaling cascade independent of their ionotropic signaling.
Kainate receptors are composed of a distinct array of subunits. The subunits GluK1 to GluK3 coassemble with GluK4 or GluK5 subunits to form functional kainate receptors. Homomeric GluK1, GluK2, and GluK3 receptors expressed in mammalian cell lines bind kainate with an affinity of approximately 80 to 100 nM. These homomeric receptors may correspond to low-affinity kainate binding sites previously identified in membrane fractions of the brain. In contrast, homomeric GluK4 receptors bind kainate with an affinity of 4 nM and may correspond to high-affinity kainate binding sites in the brain. However, when they are expressed alone, GluK4 and GluK5 are virtually inactive because they lack functional channels. Consequently, it is believed that they serve as modulatory subunits that confer high-affinity kainate binding on channels formed by GluK1 to GluK3. GluK1 and GluK2 exist in several splice variants, and also undergo Q/R editing, meaning that kainate channels can vary in their Ca2+ permeability.
Selective kainate receptor antagonists are under development; those currently available, such as LY382884 5–1, are primarily selective for the GluK1 subunit. LU97175 generally antagonizes kainate receptors, although it is most potent for GluK3-containing receptors. SYM 2081 is potent agonist for GluK1- and GluK2-containing kainate receptors, and rapidly desensitizes the receptors; it can therefore be used as an antagonist. However, GYKI 53655 remains the most valuable agent for determining whether a given synaptic current is mediated by AMPA or kainate receptors, as stated earlier. Domoic acid, derived from red algae and implicated as a cause of shellfish poisoning, acts as a neurotoxin via activation of kainate receptors.
Kainate receptors are believed to contribute to the development of temporal lobe epilepsy, and genetic studies have linked alleles of GluK2 to Huntington disease (in which it might be a disease modifier). However, the role of kainate receptors in normal neuronal function, let alone in the development of complex neuropathologies, remains uncertain.
NMDA receptors have several properties that set them apart from other ligand-gated receptors. At membrane potentials more negative than approximately −50 mV, the Mg2+ in the extracellular fluid of the brain virtually abolishes ion flux through NMDA receptor channels, even in the presence of glutamate. Thus, at the resting membrane potentials that are typical of most neurons, approximately −60 to −70 mV, the activation of these receptors results in little current flow. This is because the entry of Mg2+ into the channel pore blocks the movement of monovalent ions across the channel.
In the presence of Mg2+ ions, the receptor’s current–voltage relationship has a region of slope negativity that produces a characteristic J shape when plotted (see 5–2). As the receptor’s membrane potential becomes less negative (more depolarized), the affinity of Mg2+ for its binding site decreases, and the blocking action of Mg2+ becomes ineffective; consequently, ionic current can pass through the channel as the cell membrane depolarizes.
As previously mentioned, NMDA receptors can be thought of as coincidence detectors capable of sensing simultaneous activity at a number of adjacent synapses (see 5–3). They possess this capability because they function (ie, pass current) only when they are stimulated by presynaptically released glutamate at a time when the postsynaptic cell is depolarized. Repetitive stimulation is often required because the depolarization produced by single inputs is not sufficient to relieve the blockage of the NMDA receptor channel by Mg2+.
The activation of NMDA receptors, like that of AMPA receptors, produces a nonspecific increase in permeability to the monovalent cations Na+ and K+. However, unlike most AMPA and kainate receptors in the adult CNS, NMDA receptors are highly permeable to Ca2+. Although their activation results in appreciable current and tends to depolarize the cell membrane toward the threshold for action potential firing, such activity is unlikely to represent the primary function of these receptors. Instead, NMDA receptors likely provide one of the most significant mechanisms by which synaptic activity can increase the level of intracellular Ca2+ at individual synapses. The major endogenous agonist for NMDA receptors is glutamate itself, although there is some evidence that aspartate can also activate this receptor. Numerous competitive antagonists of the agonist recognition site are available, notably D-2-amino-5-phosphonopentanoic acid (AP-5 or APV) and 3-(2-carboxypiperazin-4-yl)-1-propenyl-1-phosphonic acid (CPP). These compounds are not useful clinically because they are polar and penetrate the blood–brain barrier poorly.
The NMDA receptor is unique among all neurotransmitter receptors in that its activation requires the simultaneous binding of two different agonists. In addition to the binding of glutamate at the conventional agonist-binding site, the binding of glycine appears to be required for receptor activation 5–5. Because neither of these agonists alone can open this ion channel, glutamate and glycine are referred to as coagonists of the NMDA receptor. The physiologic significance of the glycine-binding site is unclear; however, there is some evidence to suggest that D–serine may be the endogenous agonist for this site. D-Serine, made through the conversion of L-serine by serine racemase, is subject to regulated release and specific reuptake primarily from glial cells. Thus, the glial environment of neurons may have a critical influence on NMDA receptor synaptic function.
Regardless of its physiologic role, the glycine site on the NMDA receptor may prove to be an important drug target. Cycloserine, originally developed as an antitubercular drug, is a weak partial agonist at this site and thus can modulate NMDA receptor function in vitro and in vivo. Cycloserine is reported to enhance the effects of antipsychotic drugs in patients with schizophrenia. More specific agonists, such as HA966, have been used in laboratory animals but are not yet available for clinical investigation. Derivatives of kynurenic acid, such as 5,7-dichlorokynurenic acid (5,7-DCK) and quinolinecarboxylic acid, are also competitive antagonists at the glycine site. It is important to note that the glycine site on NMDA receptors is distinct from the strychnine-sensitive glycine receptor, which mediates the independent neurotransmitter functions of glycine (see below).
Another important site on the NMDA glutamate receptor binds phencyclidine (PCP) and related drugs such as MK801 and ketamine 5–5. These drugs, which bind at or near the Mg2+-binding site, occlude the NMDA receptor channel. Thus, they act as noncompetitive receptor antagonists, and their actions, like those of Mg2+, are somewhat voltage dependent. These drugs exert potent effects on the brain. At higher concentrations they are psychotomimetic and produce effects, such as cognitive impairment, hallucinations, and delusions that are similar to some of the symptoms of schizophrenia (Chapter 17). These effects have led to the hypothesis that schizophrenia may involve hypofunction of NMDA receptors, which has in turn prompted the development of drugs that block the reuptake of glycine for the treatment of schizophrenia. The idea is that glycine transporter-1 (GlyT1) inhibitors would increase levels of extracellular glycine in forebrain and thereby facilitate activation of NMDA receptors. At still higher doses, drugs such as PCP and ketamine are dissociative anesthetics, used primarily in veterinary and pediatric practice. Interestingly, doses of ethanol associated with the upper range of intoxication in humans exert effects on NMDA receptors that are similar to those produced by PCP, ketamine, and related drugs. More recent research has shown that lower doses of ketamine, devoid of most psychotomimetic effects, exert rapidly acting antidepressant effects in a subset of individuals with treatment-resistant depression (Chapter 15).
NMDA receptors also have one or more modulatory sites that bind polyamines. The occupancy of one of these sites relieves tonic proton block and thereby potentiates NMDA receptor activation. At higher concentrations, however, polyamines act on an extracellular site to produce a voltage-dependent block of the ion channel and consequently inhibit receptor activation. Many additional drugs, such as amantadine, dextromethorphan, and memantine (Chapter 18), are weak antagonists of NMDA receptors, although it remains uncertain as to whether such actions contribute to the clinical utility of these medications.
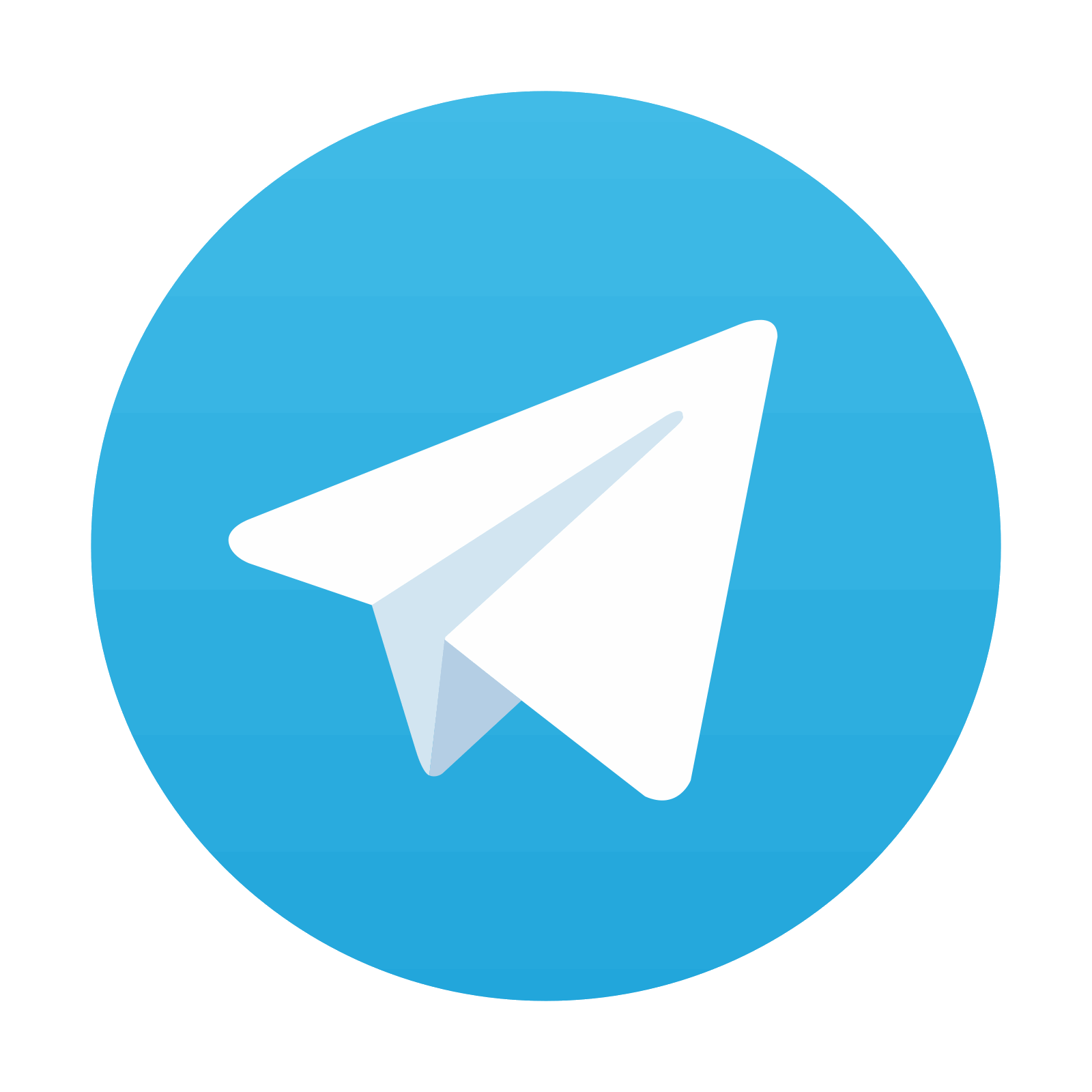
Stay updated, free articles. Join our Telegram channel
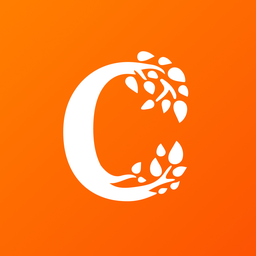
Full access? Get Clinical Tree
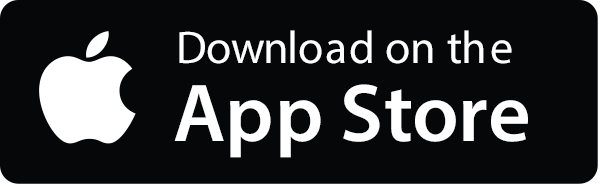
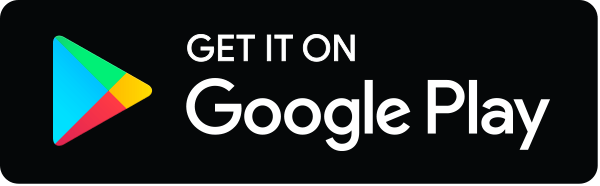