Abstract
Burst stimulation was developed based on a very simple principle that mimicking naturally occurring firing patterns might be beneficial for neuromodulation. Its main benefit lies in the capacity of burst stimulation to modulate the salience (behavioral relevance) of any stimulus, which is clinically expressed as (un)pleasantness or suffering and physiologically by the medial pain pathway. Behaviorally relevant stimuli focus the attention paid to the stimulus. Burst stimulation, in contrast to tonic stimulation, modulates not only the painfulness/loudness of a pain/sound stimulus and the descending inhibitory pain/sound system but also the suffering attached to it. Furthermore, it is capable of inducing pain suppression without the mandatory presence of paresthesia.
Keywords
Brain, Burst stimulation, Firing patterns, Neuromodulation, Spinal cord
Outline
Historical Context of Burst Stimulation 147
What Is so Special About Burst Firing in the Brain? 148
Burst Stimulation of Auditory Cortex for Tinnitus 150
Burst Stimulation for Pain 150
Working MOA of Burst Stimulation 153
What Stimulation Parameters Are Important for Pain Suppression? 153
Burst Frequency 153
Spike Frequency 154
Pulse Width 154
Number of Pulses 154
Amplitude 154
Interspike Interval 154
Total Charge 155
Is Burst Stimulation Applicable to the Entire Nervous System? 155
Future Trends 155
Conclusion 156
References 156
Historical Context of Burst Stimulation
The late Antoni Gaudi, Catalonia’s most famous architect, stated that “the architect of the future shall construct by mimicking nature because it is a more rational, longer lasting, and more economical method (of building).” By applying the same philosophy to neuromodulation, it was proposed that mimicking different firing patterns that exist in the brain in an electronic way would be a rational way of performing neuromodulation ( ).
The nervous system uses at least two firing patterns to transmit information, tonic and burst firing (see Fig. 14.1 ), and these firing patterns can be regarded as either two languages or two different components of one language. The current implanted pulse generators are capable of delivering only tonic charge-balanced stimuli, mimicking tonic firing in the brain. However, some neurons can also fire in bursts, that is, using trains of high-frequency action potentials (APs) that occur during a plateau or active phase, followed by a period of relative quiescence, called the silent phase ( ). The plateau is generated by calcium influx via T channels, on which sodium spikes ride ( ).

Burst firing has some interesting neurophysiologic properties that might be beneficial for neuromodulation purposes. Burst stimulation has therefore been developed as a novel stimulation design that should be physiologically differentiated from clustered tonic stimulation, in which every pulse is immediately charge balanced (see Fig. 14.2 ).

What Is so Special About Burst Firing in the Brain?
Burst firing can exert a strong positive ( ) or negative ( ) effect on postsynaptic responses that generates a larger excitatory or inhibitory postsynaptic potential than tonic stimulation. It has, therefore, been considered a wakeup call from the thalamus, signaling to the cortex that an important change has occurred in the sensory environment ( ). Based on this idea, it had been suggested that burst firing is capable of overriding ongoing tonic firing ( ). This is intrinsically related to the high-frequency sodium spikes that ride on a calcium plateau, mediated by T-type calcium channels. The high-frequency, closely spaced spikes in a burst cause a nonlinear buildup of the postsynaptic APs, creating a kind of super-AP ( ).
Burst firing is characteristic of unmyelinated C-fibers, a bodywide system of salience (behavioral relevance)-detecting fibers, important in hedonic homeostasis. Behavioral relevance is achieved by adding weight to an external or internal stimulus, which phenomenologically is expressed as feelings of unpleasantness (mediated through high-threshold pain C-fibers) and pleasantness ( ) (mediated through low- threshold tactile C-fibers) ( ) ( Table 14.1 ). Behavioral relevance of the pain signal is processed by a group of brain areas that used to be called the pain matrix, but are now generally accepted as a multimodal (i.e., non–pain specific (auditory, visual, somatosensory, nociceptive system), salience network) ( ), encompassing the dorsal, anterior cingulate cortex, anterior insula, dorsomedial thalamus, amygdala, hypothalamus, and periaqueductal gray ( ). This network picks up behaviorally relevant changes that occur in the internal or external environment and signals its salience to the cortex via burst firing, whereas the content of the detected change is transmitted in parallel via the modality specific pathways in tonic mode ( ).
Pain C-Fibers | Tactile C-Fibers | |
---|---|---|
Threshold | High (>2.5 mN) | Low (0.3–2.5 mN) |
Pinch, stab | Caress | |
Motivational capacity | Unpleasantness of pain | Pleasantness of caress |
Location | Hairy and glabrous skin | Hairy skin, not genitals |
Firing pattern | Burst | Burst |
Cortical activation | Anterior cingulate, anterior insula | Posterior insula |
Burst firing has some characteristics that help perform the task of a wakeup call. First, burst firing has a higher signal-to-noise ratio than tonic firing ( ), and it permits selective routing and multiplexing of information via separate pathways ( ). Selective routing and multiplexing of information have been linked to recruitment of functionally connected areas through dendrodendritic transmission of subthreshold, calcium-mediated oscillations via gap junctions ( ) on which sodium spikes can subsequently ride. This creates synchrony in segregated spatially restricted but functionally connected areas ( ).
The same burst of APs can be resonant for some neurons and nonresonant for others, depending on their natural frequencies ( ). Neither presynaptic nor postsynaptic neurons “choose” their frequencies “at will” but, instead, the frequencies are determined by the intrinsic properties of the neurons and the overall activity of the brain. Consequently, changing the frequency content of bursts and subthreshold oscillations permits the brain to determine which areas communicate with each other at any particular moment. This mechanism allows the brain to reorganize itself dynamically within a few milliseconds, without changing synaptic hardware ( ). Furthermore, the amount of spikes within a burst might be important for the nonlinear buildup of the postsynaptic potential (the wakeup call), but it is of no importance for the selective routing of information, as a nonresonant synapse will be nonresonant regardless of the amount of spikes within a burst ( ).
Moreover, burst firing at a cellular level is related to phase-amplitude coupling ( ) (i.e., cross-frequency coupling ( ), also known as nesting) at a macroscopic level. In the brain, lower oscillation frequencies such as δ (1–3 Hz), θ (4–7 Hz) or α (8–12 Hz) are transmitted over long distances, whereas higher frequencies such as β (12–30 Hz) or γ (>30 Hz) are limited to spatially restricted areas ( ). In phase-amplitude cross-frequency coupling, the amplitude of the higher nested frequency (β, γ) is related to the phase of a lower frequency (δ, θ, and α) ( Fig. 14.3 ). As such, the lower frequency functions as a carrier wave for the higher frequency ( ). The information is encoded by the higher frequency, which is broadcast throughout the brain by riding on the lower carrier frequency. Cross-frequency coupling is involved in attention ( ), emotion ( ), cognition ( ), and disease states such as pain ( ), tinnitus, Parkinson disease, depression, etc. (i.e., thalamocortical dysrhythmias) ( ).

Burst Stimulation of Auditory Cortex for Tinnitus
Burst stimulation was initially developed to treat tinnitus via the implantation of electrodes overlying the primary and secondary auditory cortices. It was assumed that burst stimulation could suppress both tonic and burst firing due to its nonlinear buildup of APs ( ), whereas tonic stimulation is be able to suppress only tonic firing (except at high frequencies by creating neurophysiologic silence) ( ).
With regard to tinnitus, it was hypothesized that noiselike tinnitus may be caused by increased burst firing in the nontonotopic (extralemniscal, nonspecific, salience) system, whereas pure tone tinnitus may be the result of increased tonic firing in the tonotopic (lemniscal, specific) system ( ). Narrow band tinnitus could be the result of a coactivation of both pathways.
When θ burst stimulation ( ) was introduced as a novel form of transcranial magnetic stimulation (TMS), it was quickly applied to tinnitus as well ( ) and extended to α and β burst TMS ( ). Compatible with the hypothesis that noise-like tinnitus was the result of increased activity in the nontonotopic, extralemniscal system (which fires in burst mode), burst TMS of the secondary auditory cortex seemed capable of suppressing noise-like tinnitus significantly better than tonic TMS ( ). Concomitant with the TMS results, a custom-made program was developed capable of creating burst stimulation in a commercially available internal pulse generator, and tinnitus patients were implanted with extradural electrodes overlying the primary and secondary auditory cortices. In an initial study, it was shown that tonic stimulation could improve only pure tone tinnitus, not noise-like tinnitus ( ).
To investigate the differential effect of burst stimulation compared with tonic stimulation, implanted tinnitus patients presenting intractable tinnitus to tonic stimulation were subsequently investigated by using burst auditory cortex stimulation ( ). Applying burst stimulation generated improvement for 48% of the tinnitus patients who did not respond to tonic stimulation ( ). Furthermore, 50% of patients who did respond to tonic stimulation obtained even more tinnitus reduction by switching to burst stimulation. In this group of responders to tonic stimulation, tinnitus suppression was 24% with tonic versus 53% for burst stimulation ( ). Therefore, adding burst stimulation improves auditory cortex stimulation for tinnitus from a poor response rate to an acceptable, though far from ideal, suppression rate ( ). Burst stimulation seems especially beneficial for patients with difficult-to-treat noise-like tinnitus ( ).
Burst Stimulation for Pain
Pain can be defined as perception that encompasses painfulness (sensory component) and suffering (affective component). Physiologically, nociceptive pain (acute pain induced by activating nociceptors) can be considered as a protective sense against a harsh environment but loses this function and becomes independent of it in chronic neuropathic pain ( ), which is defined as pain arising as a direct consequence of a lesion or disease affecting the somatosensory nervous system ( ). The unpleasantness of pain is motivational in that it induces the animal/human to do something about the pain—to orient behavior to withdraw from the painful stimulus (fight or flight).
Pain is usually an aversive signal processed by at least three pathways: two, ascending, pain-evoking pathways ( ) and at least one, descending, pain-inhibitory pathway ( ). The medial pain pathway encodes the motivational/affective component of pain ( ) (i.e., the unpleasantness) ( ), the lateral pathway encodes the discriminatory/sensory ( ) component, and the descending pathway suppresses ongoing pain in a state-dependent manner ( ). The ascending medial and lateral pain pathways are processed in parallel ( ) and can be individually modified without affecting the other pathway ( ). The ascending medial system is activated by C-fibers and connects to the mediodorsal and ventral, posterolateral nuclei of the thalamus, and from there, its connections, respectively, reach the anterior cingulate and anterior insula ( ). In fact, it is a nonspecific, salience pathway ( ), indicating the stimulus is behaviorally important. The medial pathway is activated not only by nociceptive stimuli but also by visual, auditory, and nonnociceptive somatosensory stimuli ( ). The ascending, lateral pain pathway is activated by C-, Aδ-, and Aβ-fibers and connects to the ventral, posterolateral nuclei of the thalamus and from there reaches the somatosensory cortex and parietal area ( ). The descending pain inhibitory system involves the rostral and pregenual anterior cingulate cortex and connects to the periaqueductal gray, and from there is relayed further to the somatosensory periphery ( ) ( Fig. 14.2 ).
Based on the promising results in tinnitus, it has been hypothesized that pain could be the analogue of pure tone tinnitus and that paresthesias could be the analogue of noise-like tinnitus( ). Based on the similarities between tinnitus and pain, the burst stimulation protocol was also applied to the somatosensory system, more specifically to the spinal cord and somatosensory cortex ( ).
Spinal cord stimulation (SCS) was developed more than 50 years ago as a novel treatment for medically intractable, chronic pain, targeting failed back surgery syndrome, arachnoiditis, and complex regional pain syndrome ( ), as well as chronic critical limb ischemia ( ) and refractory angina ( ). SCS not only reduces pain but also improves quality of life, reduces analgesia intake, and permits some patients to return to work, with minimal side effects apart from paresthesias ( ).
The original concept of SCS was based on the gate control theory of pain ( ), which postulated that stimulation of large Aβ-fibers can inhibit pain transmission via the small unmyelinated C-fibers and thinly myelinated Aδ-fibers. The working mechanism of action (MOA) of SCS has not been fully elucidated but very likely involves both a local effect and a combination of local spinal as well as supraspinal mechanisms ( ). At the spinal level, the ascending dorsal column fibers, as well as the opioidergic ( ), serotoninergic ( ) and dopaminergic ( ) descending pain modulatory systems, might be implicated in the pain-suppressing effect of SCS. See Chapter 15 for a thorough discussion of the MOA of SCS.
Since the initial conception of the pain gate theory, it has been shown that when larger myelinated fibers degenerate, the high-threshold, unmyelinated, pain-transmitting C-fibers, but not the low-threshold, tactile C-fibers, start firing spontaneously in rhythmic bursts, which is related to the pain ( ). Thus, it intuitively makes sense that electrically stimulating the remaining large Aβ-fibers might suppress this spontaneous, pain-related, rhythmic bursting.
The unique feature of burst technology is related to its fundamental aspect of mimicking nature, more specifically the burst firing pattern that exists in our nervous system from the periphery, to the autonomic nervous system, to the spinal cord, all the way up to the brain, both in the reward system and in the thalamocortical columns. By providing stimulation in a physiologic manner, burst stimulation permits paresthesia-free SCS ( ). Indeed, there is no reason why, in a physiologic way, paresthesia would have to be required to obtain pain suppression, as is the case with tonic stimulation ( ).
The beauty of a paresthesia-free, stimulation experiment (as with burst SCS) is that 40 years after its inception ( ), for the first time, a truly randomized, double-blinded, placebo-controlled study can be performed, comparing active SCS (no paresthesia) with sham SCS (no paresthesia) and finally demonstrating what every pain physician already knows—that SCS is better than placebo for all pain measures (back, limb, and global pain) ( ).
Whereas this paresthesia-free stimulation (Burst SCS) ( ) might seem important for patient satisfaction, a recent study demonstrated that this was a reason to prefer burst technology over tonic stimulation for only 10% of patients ( ). What is more important for patient satisfaction is the fact that burst stimulation modulates the affective and attentional components of pain. This is demonstrated by the dramatic score differences in the pain vigilance and awareness questionnaire, which measures attention to pain and changes in pain, between patients in the burst SCS group and patients in the tonic SCS group ( ). Defocusing the patient’s attention from pain is similar to what frontal lobotomies evoke ( ). It is of interest that the area that burst stimuli modulates is the same area where cingulotomies (i.e., dorsal anterior cingulate cortex [dACC]) ( ) were and are performed ( ). This dACC area colocalizes with the area that encodes the unpleasantness of pain ( ), the behavioral relevance of pain (i.e., salience) ( ), and the emotional and suffering aspects of pain ( ). The dACC modulation by burst stimulation also significantly differs from the area that tonic stimulation modulates ( ). From an electrophysiologic point of view, burst SCS modulates the dACC ( ), which is the end station of the medial pain system ( ) ( Fig. 14.4 , Table 14.2 ). This is most likely why 80–90% of people who are treated with both burst and tonic stimulation prefer burst stimulation ( ).

Burst Stimulation | Tonic Stimulation |
---|---|
Descending pain inhibitory (pgACC, PHC) | Descending pain inhibitory |
Lateral pain pathway (SSC) | Lateral pain pathway |
Nonlinear stronger activator | |
Medial pain pathway (rACC/DLPFC) | – |
Rerouting/multiplexing | |
Normalizes pain promoting/pain suppressing balance | Decreases pain promoting/pain suppressing balance |
Dopamine? | GABA |
For a similar amount of discriminatory/sensory pain suppression, the affective, attentional component of pain is better suppressed, so that patients do not care about their pain anymore; they can defocus from their pain. This is why, even if limb pain and back pain, in a placebo-controlled study, were not better suppressed by burst SCS than with tonic SCS, global pain was better suppressed with the burst technology ( ). Global pain perception can be seen as the combination of painfulness and suffering; that is, it combines the discriminatory/sensory component of pain (spatial, type, intensity) and the affective/motivational component of pain, whereas a visual analog scale or numeric rating scale of limb and back pain does not. It is important to note, however, that these initial results comparing burst SCS with tonic SCS were comparing results at very short time periods of stimulation (1–2 weeks), using different stimulation designs, and that, in longer follow-up periods, all measures become superior with burst technology ( ). The reason for this time course of difference is currently unknown, but it can be hypothesized that, through its attentional neglect for the pain, pain intensity also progressively decreases.
A study that more specifically looked at what kind of pain was better suppressed with burst technology demonstrated that neuropathic pain is better suppressed than failed back surgery pain ( ).
Another interesting finding is that increasing the frequency above 500-Hz spike mode does not seem to elicit a further improvement in pain suppression. Indeed, a study demonstrated that 500- and 1000-Hz burst stimulations do not significantly differ in pain suppression ( ), creating doubt as to the extra value of driving stimulation devices to very high frequency stimulation ( ). Indeed, in a study comparing burst SCS versus 10-kHz stimulation, back pain was equally well suppressed, and burst stimulation seemed to better suppress limb pain ( ). Interestingly, burst and 10-kHz stimulations improve mood and sleep equally well ( ).
Working MOA of Burst Stimulation
The exact working MOA of burst stimulation is incompletely unraveled. Theoretical assumptions based on the physiologic function of burst firing in both animal and human studies have proposed multiple, scientifically testable hypotheses and working MOAs, which are not mutually exclusive ( Tables 14.2 and 14.3 ).
Hypothetical Working Mechanisms of Burst Stimulation |
---|
|
Source localized EEG functional imaging data ( ) do strongly suggest that burst stimulation not only modulates the lateral and descending pain pathways but also has an influence on the medial pain pathway, as the dorsal part of the ACC is modulated by burst stimulation when contrasted to tonic stimulation. This extra modulation of the medial pain pathway by burst stimulation, while also modulating the descending, pain inhibitory and ascending, lateral pain pathway, is possibly due to the following MOAs:
- 1.
Burst firing has routing and multiplexing capacities ( ).
- 2.
Burst stimulation is a stronger activator ( ) and inhibitor ( ) of postsynaptic potentials than tonic stimulation.
- 3.
The use of 500 Hz in the burst mode has a maximal inhibitory effect on the postsynaptic neuron ( ).
- 4.
Burst firing, at 500 Hz, like high-frequency firing, could selectively modulate Aβ-fibers, without activating C-fibers ( ).
- 5.
Burst stimulation modulates low-threshold, tactile C-fibers, which are known to be antinociceptive ( ).
- 6.
Burst stimulation induces frequency-dependent opioid release from dorsal horn (DH) neurons with a maximal release at 500 Hz ( ).
- 7.
Burst stimulation could modulate electrical gap junctions in the DH, which are known to be involved in the production of chronic pain ( ), as burst firing can induce activity-dependent, long-term depression of these electrical synapses ( ).
Looking for these alternative working MOAs is important as it is clear from animal research that burst stimulation does not exert its pain-inhibitory effect through GABAergic mechanisms, as does tonic simulation ( ).
What Stimulation Parameters Are Important for Pain Suppression?
In contrast to tonic stimulation, the parameter space of burst stimulation is very large. Whereas for tonic stimulation, there are only three parameters that can be modified (frequency, pulse width, and amplitude, there are six parameters that can be adjusted for burst stimulation, creating both an unseen freedom of programming and a possible nightmare, if programming without knowledge.
Burst Frequency
For burst SCS, the default setting consists of 40-Hz burst mode, with five spikes at 500-Hz spike frequency, a pulse width of 1000 μs, and an interspike interval of 1000 μs, which is based on thalamic burst parameters. The 40-Hz burst mode is similar to the frequency that is most commonly used in tonic SCS. Whether burst frequency is important in humans is unknown. An animal pain model study of burst SCS did not find any significant differences in the effectiveness of burst SCS, either for the amount of responding neurons or in reduction of neuronal activity for different burst frequencies ( ). However, whether this translates into clinical experience has yet to be determined.
Based on the principle that therapeutic burst stimulation should mimic physiologic burst firing patterns in the nervous system, it is conceivable that burst firing in different parts of the nervous system would require different parameters. For example, burst firing in the reward system and autonomic nervous system in humans is characterized by low-frequency bursts in the δ–θ frequency range ( ). In clinical practice, burst stimulation at the cingulate gyrus ( ) seems to be most efficacious at 6–10 Hz burst mode and at the primary somatosensory cortex at 4–8 Hz burst mode ( ), whereas burst stimulation at the posterior part of the superior temporal gyrus (i.e., secondary or tertiary auditory cortex) seems to be more variable ( ). However, no systematic study has been undertaken to verify whether this assumption is correct.
Spike Frequency
An animal study has demonstrated that spike frequency is important in determining the amount of responsive neurons to burst SCS ( ); however, spike frequency of burst SCS does not influence neuronal activity ( ). 500 Hz is selected for the reasons discussed here. As mentioned, the burst-firing sodium spikes ride on a calcium-dependent plateau. The positive deflection of the plateau and positive spikes are charge balanced after all high-frequency spikes are terminated (i.e., at the end of the entire burst). This differentiates burst stimulation from high-frequency clustered tonic firing, in which all spikes within the burst are individually charge balanced. A recent study demonstrates that there is a clinical difference when comparing continuous 500-Hz subthreshold tonic mode with 500-Hz burst mode ( ), demonstrating the importance of the burst waveform design for clinical efficacy.
Pulse Width
In contrast to activating axons, which can be accomplished by using pulse widths of up to 300 μs, a 1000-μs pulse width is required if the aim is to be as physiologic as possible (i.e., to activate dendrites and cell bodies) ( ). In a recent animal study, it was shown that there exists a pulse width–dependent suppression of pinch evoked firing rates. Pulse widths of <500 μs increased pinch evoked firing, whereas pulse widths of >500 μs decreased pinch evoked firing rates. Pulse widths of 500 μs resulted in a 15% decrease of pinch evoked firing rate, whereas pulse widths of 750 and 1000 μs produced a 24% and 49% decrease, respectively ( ). Furthermore, in bursting dopaminergic neurons, which signal salience, there is a pulse width–dependent increase in dopamine release, signifying more salience as more dopamine is released, with a maximal release at 1000 μs ( ).
Number of Pulses
In burst firing, the probability of inducing a postsynaptic potential is determined by the amount of pulses within a burst ( ). The effectiveness of signal transmission follows a non-linear curve and adding more pulses beyond five does not add much to effectiveness. That the number of pulses is not only important in burst firing but also in burst SCS is confirmed in an animal study ( ). Whereas the number of pulses does not seem to determine the amount of cells that respond to burst SCS, it does influence neuronal activity, with more reduction obtained by a higher number of pulses ( ).
Amplitude
The amplitude of the stimulation is important for the efficacy of burst SCS, as an animal study has demonstrated, both for reducing neuronal activity and for increasing the amount of responding neurons ( ). In humans, no specific study has been performed to evaluate the importance of amplitude. However, the first clinical data suggest that low amplitudes suffice for obtaining pain suppression and that paresthesia, which is related to amplitude, is not required to obtain a beneficial effect ( ). An intriguing question is whether this pain-suppressive effect could be nonlinear (i.e., follow an inverted-U curve), where low-intensity and high-intensity burst SCS could yield the same clinical effect. If so, an intermediate amplitude would be optimum. The reason for this question lies in the fact that activation of the dACC, which is modulated by burst but not tonic stimulation, follows an inverted-U shape ( ). Indeed, a clinical study suggests that there is group of patients who prefer high-amplitude stimulation and a group who prefer low-amplitude burst SCS to control their neuropathic pain ( ).
Interspike Interval
The importance of the interspike interval has not been scientifically investigated, in either animal or human studies. It is theoretically possible that the interspike interval has some influence on the nonlinear buildup of the postsynaptic potential, but data are lacking to support or refute this mechanism.
Total Charge
Whereas the initial pilot study on burst SCS suggested that burst stimulation was delivering more charge to the spinal cord ( ), it is questionable whether charge is of clinical importance in human burst SCS, even though an animal study with burst SCS does suggest so ( ). Indeed, burst SCS is now routinely applied in cycle mode in a 1:2 to 1:3 ON:OFF protocol, which clinically suggests that the charge or energy delivery per second to the spinal cord might not be a determining factor for clinical efficacy but merely for generating APs.
Historical Context of Burst Stimulation
The late Antoni Gaudi, Catalonia’s most famous architect, stated that “the architect of the future shall construct by mimicking nature because it is a more rational, longer lasting, and more economical method (of building).” By applying the same philosophy to neuromodulation, it was proposed that mimicking different firing patterns that exist in the brain in an electronic way would be a rational way of performing neuromodulation ( ).
The nervous system uses at least two firing patterns to transmit information, tonic and burst firing (see Fig. 14.1 ), and these firing patterns can be regarded as either two languages or two different components of one language. The current implanted pulse generators are capable of delivering only tonic charge-balanced stimuli, mimicking tonic firing in the brain. However, some neurons can also fire in bursts, that is, using trains of high-frequency action potentials (APs) that occur during a plateau or active phase, followed by a period of relative quiescence, called the silent phase ( ). The plateau is generated by calcium influx via T channels, on which sodium spikes ride ( ).

Burst firing has some interesting neurophysiologic properties that might be beneficial for neuromodulation purposes. Burst stimulation has therefore been developed as a novel stimulation design that should be physiologically differentiated from clustered tonic stimulation, in which every pulse is immediately charge balanced (see Fig. 14.2 ).

What Is so Special About Burst Firing in the Brain?
Burst firing can exert a strong positive ( ) or negative ( ) effect on postsynaptic responses that generates a larger excitatory or inhibitory postsynaptic potential than tonic stimulation. It has, therefore, been considered a wakeup call from the thalamus, signaling to the cortex that an important change has occurred in the sensory environment ( ). Based on this idea, it had been suggested that burst firing is capable of overriding ongoing tonic firing ( ). This is intrinsically related to the high-frequency sodium spikes that ride on a calcium plateau, mediated by T-type calcium channels. The high-frequency, closely spaced spikes in a burst cause a nonlinear buildup of the postsynaptic APs, creating a kind of super-AP ( ).
Burst firing is characteristic of unmyelinated C-fibers, a bodywide system of salience (behavioral relevance)-detecting fibers, important in hedonic homeostasis. Behavioral relevance is achieved by adding weight to an external or internal stimulus, which phenomenologically is expressed as feelings of unpleasantness (mediated through high-threshold pain C-fibers) and pleasantness ( ) (mediated through low- threshold tactile C-fibers) ( ) ( Table 14.1 ). Behavioral relevance of the pain signal is processed by a group of brain areas that used to be called the pain matrix, but are now generally accepted as a multimodal (i.e., non–pain specific (auditory, visual, somatosensory, nociceptive system), salience network) ( ), encompassing the dorsal, anterior cingulate cortex, anterior insula, dorsomedial thalamus, amygdala, hypothalamus, and periaqueductal gray ( ). This network picks up behaviorally relevant changes that occur in the internal or external environment and signals its salience to the cortex via burst firing, whereas the content of the detected change is transmitted in parallel via the modality specific pathways in tonic mode ( ).
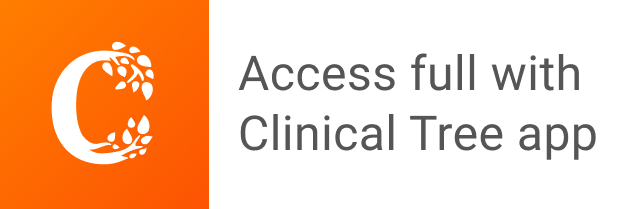