Gap Junctions
Abstract
Electrotonic coupling between cells is accomplished through the formation of gap junctions (GJs) between cells. Composed of connexin proteins, GJs are found on multiple cell types and connexin distribution is cell type-specific. All GJs play a role in cellular communication, but astrocytic GJs are thought to play a role in K+ and glutamate redistribution, synaptic strength regulation, and memory formation. Both human tissue studies and animal models of epilepsy have shown considerable changes in connexin expression after seizure activity. Electrophysiological studies have implicated GJs in the generation of very fast oscillations that precede seizures. Knockout and GJ inhibitors studies have demonstrated potential anticonvulsant effects, although these results are mixed and suffer from lack of specificity of many of the currently available GJ inhibitors. Findings implicating GJs in epilepsy as well as differences in the roles of neuronal versus glial GJs in tissue excitability are considered in this chapter.
Keywords
Gap junction; (GJ); coupling; connexin; Cx43; Cx30; carbenoxolone; mefloquine; quinine; tracer coupling
Overview
Electrotonic coupling between cells is accomplished through the formation of gap junctions (GJs) between cells. Composed of connexin proteins, GJs are found on multiple cell types and connexin distribution is cell type-specific. All GJs play a role in cellular communication, but astrocytic GJs are thought to play a role in K+ and glutamate redistribution, synaptic strength regulation, and memory formation. Both human tissue studies and animal models of epilepsy have shown considerable changes in connexin expression after seizure activity. Electrophysiological studies have implicated GJs in the generation of very fast oscillations (VFOs) that precede seizures. Knockout and GJ inhibitor studies have demonstrated potential anticonvulsant effects, although these results are mixed and suffer from lack of specificity of many of the currently available GJ inhibitors. Findings implicating GJs in epilepsy as well as differences in the roles of neuronal versus glial GJs in tissue excitability are considered in this chapter.
History
In the 1940s and 1950s, it was hypothesized that cells had a surface barrier that allowed very small molecules, like inorganic ions, to pass through membrane pores or channels. This was based on permeability studies and electrical measurements of various cell types that showed that membranes were highly diffusive [1]. Subsequently, observations using electron microscopy revealed a seemingly continuous surface of membranes in various cell types. Finally, the first evidence for an electrical connection between cells came in the late 1950s when direct electrical transmission between nerve cells in both the lobster cardiac ganglion [2] and the giant motor synapses of crayfish was reported. In the 1960s, electrotonic coupling in vertebrate tissue was discovered between teleost spinal neurons [3].
Although a likely instance of electrotonic coupling was described between spinal motoneurons of the cat in the 1960s [4], it was not truly demonstrated physiologically between neurons in the mammalian central nervous system (CNS) until the early 1970s. Electrotonic coupling between mesencephalic neurons of the fifth nerve in rat [5] and between neurons in the rat lateral vestibular nucleus [6] were observed. The hypothesis that coupling between neurons could mediate the synchronization of neurons during seizure activity was proposed after MacVicar and Dudek [7] used dual intracellular recordings in rat hippocampal slices to demonstrate direct electrotonic coupling of neurons. Shortly thereafter, three independent research groups proved that chemical synaptic transmission was not necessary for the synchronization of neuronal activity in the CA1 region of the hippocampus. This was accomplished by showing that, after incubating rat hippocampal slices in a low calcium media to block chemical synaptic transmission, prolonged bursts of large population spikes in hippocampal pyramidal cells could still occur [8–13]. Over the next several decades, the role of GJs in various models of epilepsy has been examined.
Structure
Connexins are vertebrate transmembrane proteins that assemble into groups of six to form pores called connexons or hexameric hemichannels. A complete GJ is formed by two connexons, thus connecting the cytoplasm between two cells (Fig. 11.1) [14]. These assemblies can be either homomeric or heteromeric in nature. In invertebrates, six innexin protein subunits come together to form an innexon, or hemichannel. Two apposed innexons then form GJs. To date, 20 connexin genes in the mouse genome and 21 connexin genes in the human genome have been discovered [14,15]. In 2004, another family of GJ proteins homologous to invertebrate innexins, called pannexins, were cloned [16]. Pannexins were initially suspected to form GJs due to their structural similarity to connexins, however, they predominantly form hemichannels.

Connexin 43 (Cx43) and connexin 45 (Cx45), as examples of connexin family members, typically thread through the membrane four times, with the N-terminus, C-terminus, and cytoplasmic loop exposed to the cytoplasm. Connexin arrangement in the membrane also yields two extracellular loops designated EL-1 and EL-2. Six connexins oligomerize into a connexon or hemichannel that docks in homotypic, heterotypic, and combined heterotypic/heteromeric arrangements. In total, as many as 14 different connexon arrangements can form when two members of the connexin family intermix. Source: Reproduced with permission from Laird DW. Life cycle of connexins in health and disease. Biochem J 2006;394(Pt 3):527–43.
Kanno and Loewenstein [17] discovered that fluorescein-sodium, a 376 Da hydrophilic molecule, could traverse GJs [18]. Shortly thereafter, various tracer molecules more than 1 KDa in mass were shown to pass through GJs [19]. GJs can exchange ions, energy metabolites, neurotransmitters, and signaling molecules. The large hydrophilic pore of GJs allows chemical and metabolic coupling in addition to the well-known electrical coupling. In addition to neurotransmitter release through hemichannels and cellular communication through GJs, connexins serve a variety of functions in the brain.
Cellular Distribution and Function
Hemichannels allow the exchange of ions and small signaling molecules between the cytoplasm and extracellular environment whereas GJs provide the ability for cell-to-cell communication. Connexins exhibit a cell-specific distribution (Table 11.1) and have been studied in both human tissue and animal models of epilepsy. Several studies have demonstrated a role for GJ coupling in seizure activity, although these findings have been debated. The discrepancy, in part, could be due to the functional difference between glial and neuronal GJs; therefore, both will be discussed in this chapter.
Table 11.1
Cellular Distribution of Connexin Proteins
Cell Type | Connexins Expressed |
Neurons | Cx30.2, Cx36, Cx45 |
Astrocytes | Cx26, Cx30, Cx40, Cx43, Cx47 |
Oligodendrocytes | Cx29, Cx32, Cx36, Cx47 |
Microglia | Cx43 |
Astrocytes are predominantly connected through the proteins connexin 30 (Cx30) and connexin 43 (Cx43), although their distribution is heterogeneous throughout the brain. For example, Cx30 expression is highest in the cerebellum and is significantly lower in both the hippocampus and cortex; Cx43 is predominantly responsible for coupling in the hippocampus [20] and is also expressed on activated microglia [21]. Oligodendrocytes are coupled mainly through connexin 47 (Cx47) and connexin 32 (Cx32) and play a major role in myelination [22]. Griemsmann et al. [23] characterized coupled networks between astrocytes and oligodendrocytes in the murine ventrobasal thalamus, hippocampus, and cortex of mice and found large panglial networks in all three gray matter regions. They found that Cx30 is the dominant astrocytic connexin in the thalamus and functional channels formed by Cx30 and Cx32 predominantly mediate astrocyte to oligodendrocyte coupling in the thalamus. Finally, deletion of Cx30 and Cx47 resulted in the loss of panglial coupling and this could be restored with the presence of either one of the connexin alleles [23].
Astrocytic coupling is both temperature- and anisotropy-dependent [24]. GJ networks in astrocytes are known to regulate cell volume, contribute to spatial buffering of potassium, modulate glutamatergic synaptic activity and synaptic plasticity, and play a role in information processing, sensorimotor, and spatial memory tasks [25–30]. Interestingly, Pannasch et al. [26] recently discovered a new role of the astrocytic Cx30, independent of its channel function. Cx30 can regulate the extent of astrocyte invasion into glutamatergic synapses (Fig. 11.2) [31], thus determining the efficacy of glutamate clearance and excitatory synaptic strength [26]. This agrees with previous findings that astrocytic coupling is found exclusively on “classical” astrocytes expressing glutamate transporters in the hippocampus (termed “GluT” cells by Steinhäuser and colleagues) that can help modulate the strength of glutamatergic synaptic activity; coupling was largely absent from AMPA-type glutamate receptor-bearing cells (“GluR” cells) with astroglial properties [32]. Importantly, astrocytic networks can combat K+ and glutamate hyperexcitability by removing and redistributing these excitatory molecules [27,30].
Connexin 36 (Cx36) and connexin 45 (Cx45) are the predominant proteins responsible for neuron–neuron gap junctional coupling [33,34]. In particular, Cx36 is largely expressed by interneurons [35] and appears to electrically couple hippocampal and cortical GABAergic interneurons [36]. Neuronal GJ coupling is thought to underlie synchronous neuronal activity, long-term potentiation, short-term and spatial memory formation, and motor coordination learning [35,37–40]. Importantly, electrical coupling may influence neuronal activity and has been proposed that such coupling could underlie the generation of synchronous neuronal activity [35,41]. Increasing evidence for the role of GJs in seizure initiation, epileptogenesis, and tissue synchronization has been presented in both human tissue studies and experimental models of epilepsy.
Human Tissue Studies
The first human tissue studies to examine GJ coupling in epileptic tissue were conducted in the early 1990s. Increased Cx43 and Cx32 mRNA levels were observed in resected epileptic temporal lobe tissue for the management of intractable epilepsy compared to peritumoral temporal cortex tissue [42]. In a subset of patients with tumors associated with acute seizures, even higher levels of Cx43 mRNA were observed. In contrast, however, Elisevich et al. found no upregulation in mRNA or protein levels of Cx43 in hippocampal tissue resected from patients presenting with intractable epilepsy when compared to nonepileptic tissue from patients requiring temporal lobectomy for life-threatening situations [43]. Although there was a decline in the mean levels of Cx43 mRNA expressed predominantly in astrocytes in the epileptic tissue, there was no significant change in the corresponding protein levels. The authors point out that their findings do not rule out the possibility of a change in the dynamic state (open vs closed) of the GJs playing a role in epileptogenicity.
Lee et al. used fluorescence recovery after photobleaching (FRAP) to quantify GJ coupling in tissue from patients who underwent temporal lobectomies for intractable epilepsy. Specifically, primary astrocytes were derived ex vivo from the hippocampus, hyperexcitable parahippocampus, and the “normal” cortex. This was compared to primary astrocytes derived from the cortical margins with normal EEG activity and the surrounding hyperexcitable cortex from patients who underwent craniotomy for astrocytoma resection [44]. GJ coupling was more pronounced in astrocytes isolated from the hyperexcitable tissue, as determined by faster and more complete fluorescence recovery [44].
The most comprehensive and recent study examining the functional properties of astrocytes from human epilepsy tissue specimens was by Bedner et al. [45]. These authors studied 119 specimens from patients with mesial temporal lobe epilepsy (MTLE) with (n = 75) and without (n = 44) sclerosis. They found that in MTLE specimens with typical hippocampal sclerosis, there is a complete absence of typical “classical” astrocytes and an absence of astrocyte gap junctional coupling. In contrast, coupled astrocytes were abundant in nonsclerotic hippocampus [45].
Thus far, only three studies have used immunohistochemical techniques to analyze changes in GJ protein expression in human epileptic tissue. Two studies found that hippocampal tissue resected from patients with MTLE had elevated Cx43 immunoreactivity (IR) compared to autopsy controls [46,47]. In addition, decreased levels of Cx32 IR and preservation of Cx36 IR were observed in the epileptic tissue [47]. The third study examined protein localization of Cx43 in patients with epilepsy secondary to focal cortical dysplasia (FCD) and with cryptogenic epilepsy [48]. Patients with FCD type IA and FCD type IIA, and cryptogenic epilepsy revealed a similar distribution of Cx43 IR to that of control tissue. In tissue from patients with FCD type IIB, however, Cx43 IR clustered in large aggregates of puncta around vimentin-immunopositive and CD34-immunonegative balloon cells and hypertrophic astrocytes [48]. Interestingly, no changes in Cx43 mRNA levels were found in epileptic tissue compared to control. The authors did note, however, that the Cx43 transcript levels were relatively homogenous in control tissue but highly variable in the epileptic tissue. Investigating further, the authors found a threefold increase of Cx43 mRNA levels in a subgroup (25%) of cryptogenic epilepsy specimens compared to both the control and FCD tissue [48].
A significant disadvantage to human tissue studies is the lack of appropriate controls. As a result, many studies use tumor specimens as controls for epileptic tissue (and vice versa). Several studies, however, have shown downregulation of GJ protein in tumor tissue. For example, Cx43 expression is decreased in gliomas [49–51] and a variety of breast tumors [52]. Although Aronica et al. found decreased Cx43 IR in high-grade gliomas, they also found increased Cx43 IR in reactive astrocytes in the epileptic cortex surrounding low-grade tumors [51]. This suggests that increased Cx43 levels may contribute to tumor-related seizures. In addition, decreased GJ coupling assayed by FRAP has been observed in primary astrocyte cultures derived from glioma specimens compared to epilepsy tissue [50]. Therefore, the controls used in these studies should be taken into account when considering human tissue data. A second disadvantage of human tissue studies is that they are often a representation of the end-point of a disease. To determine changes in connexin expression and gap junctional coupling during epileptogenesis, animal models must be used.
Knockout Mice Studies
Neuronal Connexins
Although mice deficient in the major neuronal connexin Cx36 showed no obvious phenotypic abnormalities, Cx36 has been implicated in a number of neurological functions and behavioral tasks. For example, Cx36 knockout mice (Cx36−/−) exhibited impaired visual transmission [53] and elevated auditory brainstem response thresholds [54]. Electrical coupling and synchronous activity among inhibitory interneurons in the neocortex [35] and neurons of the inferior olivary nucleus [55] were deficient in Cx36−/− mice but no significant change in coupling in the corpus callosum was observed [56]. Deletion of Cx36 led to the loss of functional synapses between GABAergic interneurons in hippocampal slices from adult mice [57]. Cx36 knockout mice exhibited reduced γ-frequency oscillations with sharp wave–burst discharges accompanied by population spikes, or “ripple” oscillations [57,58]. These burst discharges were abolished by carbenoxolone, a general GJ blocker [58]. In contrast, Maier et al. (2002) showed that hippocampal slices from mice lacking Cx36 had less frequent sharp waves and ripple oscillations. An in vivo electrophysiological analysis of the hippocampus revealed that fast-field ripple oscillations were present in both wild-type and Cx36−/− mice but that the power in the γ-frequency band and the magnitude of theta-phase modulation of gamma power were reduced in the knockout mice during wheel running [59].
In the striatum, interneurons provide the main inhibitory input to the principal projection medium-sized spiny neurons. Using patch clamp recordings from medium-sized spiny neurons in the striatum, Cummings et al. [60] determined that Cx36 knockout mice had reduced frequency of both excitatory and inhibitory spontaneous postsynaptic currents. Cx36 is essential for the maintenance of presynaptic inhibition, inducing the regulation of transmission from Ia muscle spindle afferents in the spinal cord [61]. This was determined by dorsal root potentials evoked by low-intensity stimulation of sensory afferents, which were reduced in amplitude and duration in Cx36 knockout mice. Additional knockout studies determined that Cx36 is also involved in long-term potentiation [39]. Recordings from behaving mice showed that spatial selectivity of hippocampal pyramidal neurons was reduced and less stable in knockout mice [38]. Mice deficient in Cx36 also exhibited slower theta oscillations, reflecting altered network activity as well as impaired short-term and spatial memory, but exhibited normal spatial reference memory [38]. In agreement with this, Zlomuzica et al. showed that Cx36−/− mice exhibited impaired one-trial object placement recognition [40]. In addition, Cx36 knockout mice demonstrated increased locomotion and running speed in the open-field test and exhibited more anxiety-like behavior in light-dark box [40]. More recently, coupling was significantly reduced, but not abolished in the thalamic reticular nucleus of Cx36 knockout mice [62].
Frisch et al. [37] showed that Cx36−/− mice had memory impairments that scale with the complexity of the stimuli presented. In contrast to the above findings, they found that the activity patterns and the exploratory- and anxiety-related responses were similar between wild-type and Cx36−/− mice [37]. Sensorimotor capacities and learning and memory processes were impaired in the knockout mice; Cx36-deficient mice displayed slower motor coordination learning in the rotarod test. After a retention interval of 24 hours, knockout mice showed habituation in an open-field test but failed to habituate in the more complex environment of the Y-maze. Interestingly, more pronounced memory impairment was found when Cx36 knockout mice were unable to recognize recently explored objects after short delays [37].
A few studies have examined the role of Cx36 in disease models. After ischemia, Cx36 knockout mice had fewer and shorter postischemic cortical spreading depolarization events, better function outcome, and decreased infarct size [63]. In the 4-aminopyridine (4-AP) model of epilepsy, Cx36 knockout mice exhibited attenuated epileptiform discharges [64]. Cx36−/− mice also had more severe seizures than wild-type mice in response to PTZ [65]. In addition, these mice showed no difference in expression levels of connexin 30.2 (Cx30.2), endothelial connexin 37 (Cx37), Cx43, Cx45, pannexin 1, pannexin 2, or GABAA receptor α1 protein levels, suggesting the increased sensitivity to PTZ was due to the lack of Cx36. In contrast, in both the low-magnesium artificial CSF (aCSF) model and aconitine (neurotoxin that increases neuronal excitability by increasing intracellular calcium levels) perfusion in low-magnesium aCSF model, neocortical slices from Cx36 knockout mice showed no difference in seizure-like events (SLE) from WT animals [66].
When examining the effect of GABAA receptor modulation on low magnesium SLE in mouse cortical slides, Voss et al. [67] found that Cx36 knockout mice had similar frequency of SLE compared to wild-type mice in response to both etomidate (augments GABAA receptors) and picrotoxin (blocks GABAA receptors). The amplitude of SLE in response to both etomidate and picrotoxin, however, was abolished in knockout mice [67]. In addition, mefloquine, a specific Cx36 blocker, did not alter the seizure-like activity in the wild-type slices, indicating that the reduction in amplitude seen in the knockout slices was likely due to preexisting compensatory changes rather than due to the lack of the interneuron GJs. These findings support the idea that Cx36 knockout mice have increased GABAergic tone as a compensation for lack of interneuron GJs and remind us to consider the possibility of compensatory mechanisms in knockout mice when looking at the literature for genetically modified animals [67].
Cx30.2 is expressed on interneurons and Cx30.2 knockout mice (Cx30.2−/−) exhibited no differences in basal excitation and excitation-inhibition balance from wild-type mice [68]. Mice deficient in the neuronal Cx45 (Cx45−/−) exhibited no difference from wild-type mice in motor learning, behavioral habituation to a novel environment, and object place recognition, although they did have impaired novel object recognition after short delays [69]. Cx45−/− mice also exhibited similar general excitability, synaptic short-time plasticity, and spontaneous high-frequency oscillations (HFOs; sharp-wave ripples) to wild-type mice. In response to bath stimulation of hippocampal slices with kainate, Cx45 mice had significantly lower γ-oscillation amplitudes in CA3, but not in CA1 subfield, and significantly larger full-width half maximum of the frequency distribution in the CA1 subfield [69].
Glial Connexins
Cx32 is highly expressed by myelinating Schwann cells in the PNS and is found on both neurons and oligodendrocytes. Mice deficient in this GJ (Cx32−/−) protein have myelination defects, display intrinsic neuronal hyperexcitability, and have impairments in inhibitory synaptic transmission [70]. Specifically, these mice exhibited separation and splitting of myelin from the axon [71], abnormally thin myelin sheaths [72,73], unusually thick periaxonal collars, and cellular bulb formations reflecting myelin degeneration-induced Schwann cell proliferation [72]. Functionally, Cx32−/− mice experienced slight conductance slowing and increased latency of muscle response after distal stimulation of the sciatic nerve [72]. In neocortical slice preparations, Cx32−/− mice had increased membrane input resistance and enhanced intrinsic excitability in neurons [73].
A reduction in GJ coupling in oligodendrocytes can lead to myelin defects that leave the brain more vulnerable to neurological disorders. Mice deficient in Cx32 were more vulnerable to brief ischemic insults [74]. Mutations in Cx32 are associated with the X-linked form of the hereditary peripheral neuropathy Charcot-Marie-Tooth (CMT) disease [71,72], which leads to muscle weakness and atrophy, areflexia, and foot deformities. The induction of human Cx32 expression protein in Cx32 knockout mice rescued the phenotype by reducing the amount of demyelination [75].
Mice deficient in the oligodendrocyte Cx47 (Cx47−/−) showed no obvious morphological or behavioral abnormalities, but the number of coupled cells was reduced by 80% [56]. In addition, ablation of Cx47 completely abolished coupling of oligodendrocytes to astrocytes [56]. Electron microscopic analysis revealed vacuolation of nerve fibers, particularly at sites of the optic nerve where myelination begins in Cx47−/− mice [76]. In addition, mutations in Cx47 are known to cause Pelizaeus-Merzbacher-like disease (PMLD), which is characterized by severe demyelination in the CNS [77]. Cx32/Cx47-double knockout mice developed severe demyelination, inflammation, and astrogliosis [22,78], all of which could be rescued by reintroducing Cx32 [22]. The deletion of both Cx32 and Cx47 in mice completely abolished coupling and caused ~10-fold increase in input resistance in the corpus callosum [56]. In addition, Cx32/Cx47-double knockout mice had an action tremor, later developed tonic seizures and sporadic convulsions, had abundant vacuolation in nerve fibers, and died within 6–10 weeks after birth [76,79].
Other connexin knockout mice revealed a variety of functional roles for connexins. Connexin 29 (Cx29) is expressed by both oligodendrocytes and myelinating Schwann cells, however, deletion of Cx29 alone did not significantly reduce the number of coupled cells in the corpus callosum in mice [56]. It is known that Cx29 is required for normal cochlear function [80], but little is known about the role of Cx29 in seizure development and spread. Connexin 26 (Cx26) is thought to be expressed in astrocytes, although this has recently been debated [81]. Knockout of Cx26 is embryonic lethal [82] and postnatal ablation of Cx26 in the ear resulted in impaired hearing [83]. Similarly, mice deficient in Cx30 also suffer from hearing impairment [84]. Cx30 knockout mice also had reduced exploratory activity in terms of rearings, but not locomotion, in the open-field and object exploration task [85]. They also showed higher open-field center avoidance and corner preference, suggesting anxiogenic behavior, but graded anxiety and rotarod performances were similar to that of wild-type [85]. Interestingly, Cx30 knockout mice experienced altered expression patterns of Cx36 and Cx32 [86].
Knockout of astrocytic Cx43 is neonatal lethal and leads to neural crest migration deficits [87–89]. Mice with astrocyte-specific ablation of Cx43 exhibited normal architecture but revealed the necessity of Cx43 in the neuron–glia interactions required for whisker-related sensory functions and plasticity [29]. These mice were insensitive to hypoxic preconditioning [90] but had increased microglial activation after acute needle stab wound in vivo [91]. Ablation of Cx43 significantly diminished the proliferation and survival of newborn cells [92]. Mice deficient in astrocytic Cx43 had oligodendrocyte–astrocyte coupling but lacked coupling to oligodendrocyte precursors [56]. In transgenic mice with deletion of the Cx43 gene in astrocytes, increased stroke volume, enhanced apoptosis, and increased inflammatory response in focal brain ischemia were observed [93]. After focal ischemia, Cx43 heterozygote mice exhibited enhanced infarct volume and apoptosis compared to wild-type mice [94,95]. Targeted postnatal inactivation of astrocytic Cx43 enhanced spreading depression [96]. Cells from Cx43 knockout mice exhibited increased reactive oxygen species (ROS)-induced astrocytic death in vitro [97].
To examine the various roles of astrocytic GJs, conditional knockout mice were created by crossing Cx43−/− mice with Cx30 knockout mice [25,27,30]. Similar to their wild-type counterpart, these double knockout mice (Cx30−/− Cx43−/−) had astrocytes with negative resting membrane potentials, time- and voltage-independent whole-cell currents, normal astrocyte morphologies, and similar cell densities. Although Cx30−/− Cx43−/− mice exhibited no gross behavioral abnormalities, the double deficiency of Cx30 and Cx43 resulted in a complete disruption of coupling [27,30]. Despite having similar synaptic contacts as wild-type mice, double knockout mice showed increased synaptic transmission, inhibitory postsynaptic currents, whole-cell currents, AMPA/NDMA ratio, and number of functional synapses. In addition, Cx30−/− Cx43−/− mice exhibited a reduction in the rheobase and paired pulse facilitation, diminished LTP and amplified LTD at CA1 synapses [27]. Taken together, these results suggest that astrocytic connexin deficiency leads to an increase in the probability of presynaptic release, an overall increased surface density of AMPA receptors (AMPARs), and the unsilencing of synapses. Although gliotransmitter release during basal neuronal activity was unaltered in the double knockout mice, increased synaptic activity was due to enhanced GLT1 and K+ current amplitudes and the inability of disconnected astrocytes to properly remove the elevated extracellular glutamate and potassium levels during neuronal activity. Astrocytes from Cx30−/− Cx43−/− mice were hypertrophic due to the ability to take up glutamate and potassium and the inability to redistribute them. Knockout mice exhibited prominent cell swelling and decreased extracellular space volume, thus enhancing synaptic activity and prolonging neuronal activation [27]. In agreement with these findings, Wallraff et al. showed that Cx30−/− Cx43−/− mice exhibited slowed potassium clearance, increased K+ accumulation during synchronized neuronal firing, and displayed a reduced threshold for the generation of epileptiform events [30].
Mice deficient in both Cx43 and Cx30 had almost no oligodendrocyte-to-astrocyte coupling [56]. In addition, these knockout mice exhibited cellular vacuolation, particularly in oligodendrocytes, reduced myelin basic protein levels in the corpus callosum and cerebellum, deficiencies in mature oligodendrocytes, impaired motor coordination and sensorimotor adaption, impaired spatial working memory, edematous astrocytes, and increased apoptosis [25]. Radial glia express Cx43 and mice lacking Cx30 and Cx43 displayed almost complete inhibition of proliferation and had a significant decline in number of radial glia-like cells and granule neurons [98]. Ablation of these GJs also reduced neurogenesis in the adult hippocampus. In a double knockout of the oligodendrocyte Cx32 and the astrocytic Cx43, animals developed white matter vacuolation without any obvious ultrasonic abnormalities in their myelin [99]. In addition, animals suffered from sensorimotor impairment seizure activity, and a progress loss of astrocytes, but not oligodendrocytes or microglia. These knockout mice suffered from early mortality around 16 weeks of age [99].
Fast Oscillation and Seizure Synchronization
Very fast oscillations (VFOs) (generally defined as ~80 to >200 Hz), or ripples, have been shown to precede the onset of seizures in both human tissue [100–102] and in vitro studies [103–105]. Interestingly, network simulations of hippocampal circuitry predicted that these ripples were GJ-dependent [106] and very few axo-axonal GJs were required to produce the appropriate oscillatory activity [107]. Both direct dye coupling between CA1 pyramidal neurons and the electrophysiological detection of axo-axonal GJs confirmed this idea [108]. These VFOs were seen in rat hippocampal slices before epileptiform bursts, were abolished by carbenoxolone, and were not dependent on chemical synaptic transmission [107,109]. Similar findings have been observed in the cat neocortex, in which VFOs were observed at the onset of seizures and the general GJ inhibitor halothane blocked both the ripples and seizure activity in vivo [110,111]. The hypothesis was further strengthened by Maex and DeShutter [112] who demonstrated that VFOs could occur spontaneously during dendritic excitation, in the absence of ectopic axon spikes, and could stop and resume spontaneously, much like a seizure.
Since the conception of this theory, several in vitro studies have supported this hypothesis. Slices from human epileptic neocortex generated spontaneous interictal discharges and preseizure VFOs [113,114]. Although reducing synaptic inhibition failed to affect the occurrence of VFOs, reducing GJ conductance abolished VFOs [114]. In support of this, VFOs in human cortical tissue in vitro could be blocked by carbenoxolone [115]. Rat neocortex slices can generate VFOs when chemical synapses are blocked [113]. γ-Frequencies (30–80 Hz) in basolateral amygdala slices generated by the application of kainic acid (KA) could be blocked by GJ inhibitors carbenoxolone, octanol, and quinine [116]. Interestingly, Hu and Agmon [117] showed that the mode of coupling affects the strength of synchrony.
In vivo, the application of carbenoxolone or quinine on the entorhinal cortex of rats decreased VFOs in the hippocampus in the intracerebroventricular pilocarpine model of epilepsy [118]. Similarly, cortical application of carbenoxolone eliminated VFOs in the rat barrel cortex after multiple-whisker stimulation [119]. Taken together, these data suggest preseizure ripples are generated by electrical coupling of neurons and GJs are involved in the synchronization of VFOs.
Computational modeling has been used to expand on the idea that gap junctional coupling can influence ripple production and tissue synchronization. Traub et al. used a model to predict that VFOs could be generated between proximal axons of cerebellar Purkinje cells if electrical coupling was present [120]. After observing VFOs in patient electrocorticography recordings prior to ictal events, Cunningham et al. [121] used a computational model to demonstrate that steadily increasing GJ conductance led to a steady increase in VFO field frequency. Similar results were shown in a model that demonstrated that an axon plexus can exhibit a distinct kind of ripples and propagation became more reliable with increasing conductance of GJs [122]. Finally, Stacey et al. showed that coupling and physiological noise initiate oscillations and then recruit neighboring tissue, thus leading to network synchrony. Of note, “epileptic” VFOs were superior at recruiting neighboring tissue [123]. Altogether, these findings support the role of electric coupling through GJs in VFOs and seizure synchronization.
Experimental Models of Epilepsy
One major advantage of experimental models of epilepsy is the ability to examine changes in GJ expression and functionality at various stages of the disease. In addition, a variety of epilepsy and seizure models exist, allowing for the study of several different epilepsies. Both KA, a neuroexcitatory amino acid, and pilocarpine, a muscarinic receptor agonist, are commonly used to induce a model of temporal lobe epilepsy (TLE) in rodents. The drug 4-AP is a potent convulsant that acts by blocking voltage-activated K+ channels. Picrotoxin is a GABAA receptor channel blocker with convulsant effects. Similarly, tetanus toxin blocks the release of inhibitory neurotransmitters across the synaptic cleft, thereby causing generalized muscular spasms. Finally, the kindling model of epilepsy involving repeated electrical stimulations is used to study the effects of repeated seizures on the brain. All of these models give insight into potential changes that occur in the pathology of human epilepsy. Many groups have hypothesized that increased GJ coupling may aid in the development of epilepsy and the spread of seizures, but evidence to support this has been mixed.
In the rat intraperitoneal kainic acid (IPKA) model of TLE, Sohl et al. found no change in Cx30, Cx32, and Cx43 mRNA or protein levels 30 days after KA administration. They did find, however, a 44% reduction in Cx36 mRNA with only a slight reduction in Cx36 protein levels [124]. Takahashi et al. found an increased Cx43 protein and reduced Cx30 protein levels 7 days after IPKA-induced status epilepticus [125]. Using the whole-cell patch technique, they also found increased dye coupling between astrocytes and significantly faster decay time kinetics in glutamate transporter-dependent currents in hippocampal brain slices from KA-treated rats [125].
A detailed, region-specific analysis of Cx30 mRNA and immunohistological changes following intracerebroventricular injections of KA in rats was conducted by Condorelli et al. [126]. Early and transient astrocytic Cx30 mRNA and protein IR were upregulated within 6 hours of injection in the cerebral cortex and the thalamus, including the lateral habenular nucleus. At 12–72 hours post-KA treatment, however, no difference in IR from control tissue was observed in those same brain regions. Cx30 mRNA was upregulated in the hypothalamus and in the medial amygdaloid nuclei by 6 hours. At 72 hours, Cx30 mRNA expression in the thalamic nuclei returned toward basal levels but, in the cerebral cortex, levels strongly decreased at 12 and 24 hours postinjection followed by recovery to control levels by 48 hours. In the hippocampus, laterodorsal and mediodorsal nuclei of the thalamus, and in the medial nucleus of the amygdala, Cx30 mRNA levels remained downregulated for the entire time course (6–72 hours), with partial recovery of hippocampal levels at 72 hours postinjection. A progressive decrease in Cx30 IR in the stratum oriens of CA3–CA4 was observed from 12–72 hours postinjection. Of note, Cx30 mRNA was upregulated in the CA3–CA4 pyramidal layer from 6 to 48 hours post-KA treatment and IR was intensely upregulated at 72 hours posttreatment. Interestingly, Cx30 mRNA, normally expressed exclusively on astrocytes, was found in neuronal cells undergoing cell death 6–48 hours after KA treatment [126]. Altogether, this study suggests brain region–specific regulation of Cx30 during the development of epilepsy.
Condorelli et al. [127] extended their previous study with a detailed analysis of mRNA levels of various connexins in response to intracerebroventricular injections of KA. No changes in Cx37, connexin 40 (Cx40), or Cx47 mRNA were observed after KA treatment in rats. From 6 to 48 hours after KA treatment, rats expressed Cx26 and Cx45 mRNA in CA3–CA4 hippocampal layers, localized over nuclei with pyknotic morphology [127]. Cx32 mRNA was upregulated in various regions up to 72 hours after injection. Cx36 was downregulated as early as 6 hours postinjection and was completely abolished by 48 hours post-KA treatment in CA3–CA4 regions of the hippocampus. Cx36 mRNA expression was not influenced by kainate treatment in either the CA1 subfield of the hippocampus or in the cerebral cortex. Cx43 was reduced in CA3–CA4 pyramidal layer after KA treatment but was persistently upregulated in the molecular layer, stratum radiatum, stratum oriens, and the hilus of the hippocampus [127]. These changes are potentially a response to increased neuronal activity or to cell damage.
The most recent and comprehensive evaluation of astrocytic gap junctional coupling in an animal model of TLE was performed by Bedner et al. [45]. In the intracortical kainic acid model of TLE, mice exhibited decreased astrocytic coupling 4–5 days postinjection and completely lacked coupling 3 and 6 months after status epilepticus in the sclerotic hippocampus (Fig. 11.3) [45]. In the nonsclerotic hippocampus, however, coupling remained intact (Fig. 11.3). Interestingly, decreased astrocyte coupling preceded apoptotic neuronal death and the onset of spontaneous seizures. Decreased GJ coupling also impaired K+ clearance 4 hours postinjection. The authors found that proinflammatory cytokines induced the uncoupling of hippocampal astrocytes in vivo [45], which agreed with similar in vitro findings that proinflammatory cytokines have an inhibitory effect on astrocytic GJ coupling [128]. To test the hypothesis that inflammation may contribute to the pathophysiology of seizures, lipopolysaccharide was injected into mice. Five days postinjection, animals exhibited reduced GJ coupling and reduced Cx43 protein levels, but unchanged Cx30 protein amounts. The uncoupling effect of lipopolysaccharide could be fully prevented with the antiinflammatory and antiepileptic drug levetiracetam [45]. In addition, uncoupling was prevented in Toll-like receptor 4 knockout mice. Taken together, these important data suggest that inflammation may contribute to rapid uncoupling of astrocytes and the uncoupling of astrocytes may be involved in epileptogenesis.

(A) Representative example showing abolished tracer coupling in sclerotic slices obtained from epileptic mice 3 months postinjection (mpi; top left). In contrast, in the contralateral hippocampus astrocytes displayed abundant gap junction coupling, resembling control conditions (bottom left). Scale bar = 100 mm. Whole-cell currents of the filled cells were elicited (left; 50 ms voltage steps ranging from −160 to +20 mV; 10 mV increments; Vhold=−80 mV). (B) Summary of tracer coupling experiments. The extent of intercellular biocytin diffusion was compared in sclerotic and nonsclerotic slices of the injected hemisphere, and in slices from the contralateral hippocampus. 3 and 6 mpi, no spread of biocytin could be detected in sclerotic slices (3 months: n = 12 biocytin-filled passive cells from six animals; 6 months: n = 6 filled passive cells, three animals). Astrocytes were still coupled in nonsclerotic hippocampal slices of the injected hemisphere (3 months: 30 ± 20.1 coupled cells, n = 11 slices, six animals; 6 months: 27.5 ± 17.8 coupled cells, n = 10 slices, five animals) and slices obtained from the contralateral hippocampus (3 months: 40.6 ± 22.5 coupled cells, n = 10 slices, six animals; 6 months: 44.9 ± 27.7 coupled cells, n = 11 slices, three animals). Nine months after status epilepticus, complete loss of cells with passive current pattern was observed in sclerotic segments of the hippocampus (n = 18 screened slices, six animals) whereas in nonsclerotic hippocampal slices ipsilateral to the injection, and in the contralateral hippocampus, astrocytes coupled to 23.8 ± 16.8 (n = 13 slices, five animals) and 29.6 ± 21 (n = 18 slices, five animals) cells, respectively. Gap junction coupling in nonsclerotic ipsilateral slices did not differ from the contralateral side. (C) Loss of SR101 uptake by astrocytes in the sclerotic hippocampus. Incubation with SR101 of slices from epileptic mice 9 mpi resulted in astrocytic labeling in the contralateral CA1 region. In the ipsilateral hippocampus, no labeled cells were detected (n = 3 slices, three animals). Scale bar = 15 mm. Source: Reproduced with permission from Bedner P, Dupper A, Hüttmann K, Muller J, Herde MK, Dublin P, et al. Astrocyte uncoupling as a cause of human temporal lobe epilepsy. Brain 2015;138(Pt 5):1208–22.
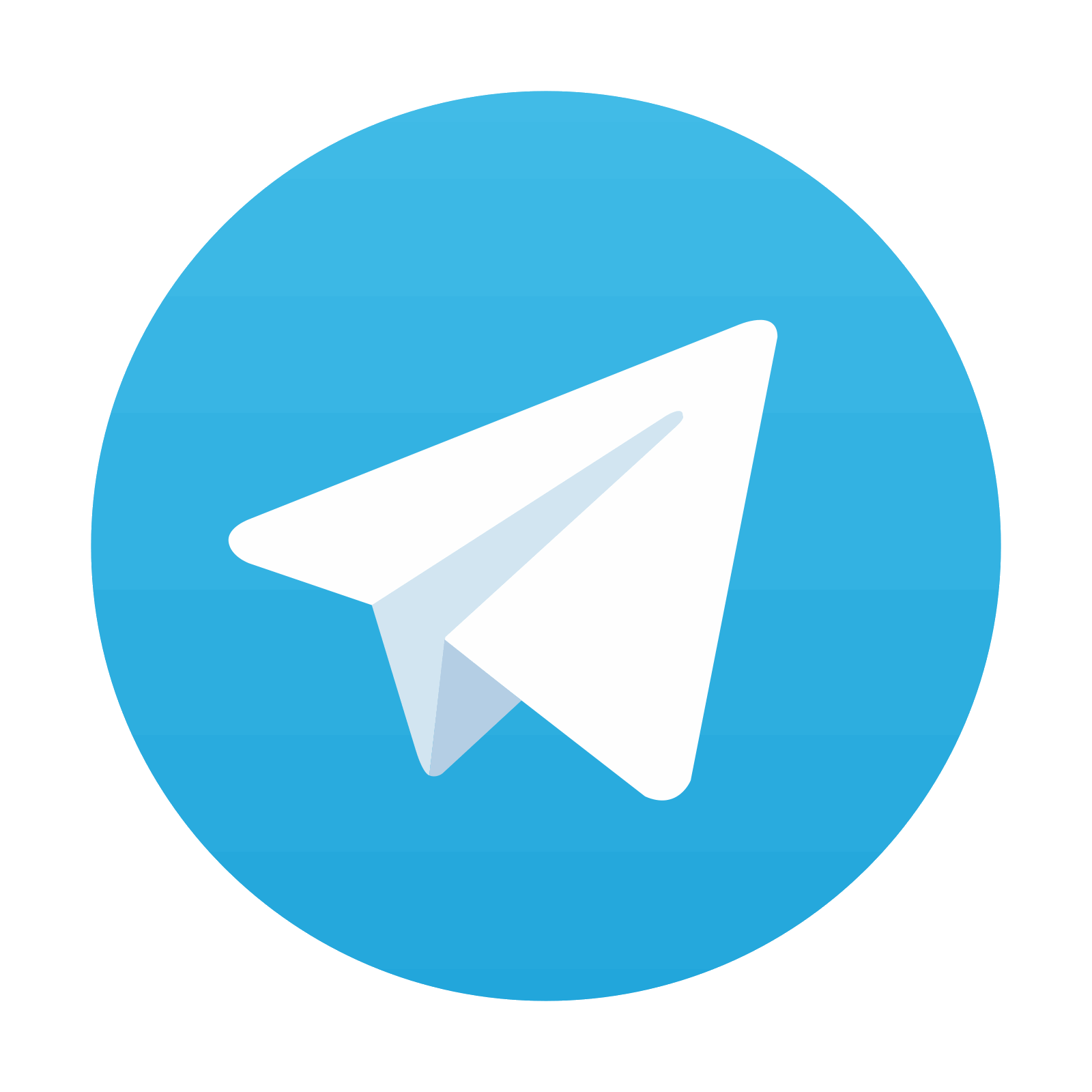
Stay updated, free articles. Join our Telegram channel
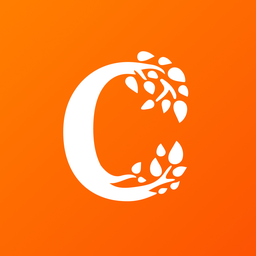
Full access? Get Clinical Tree
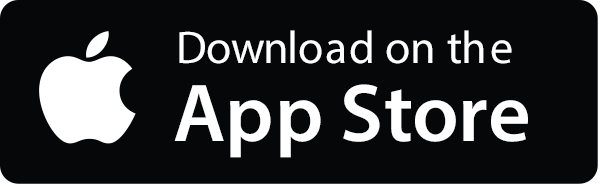
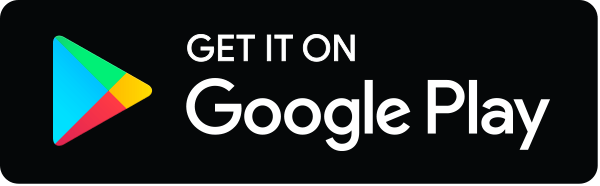
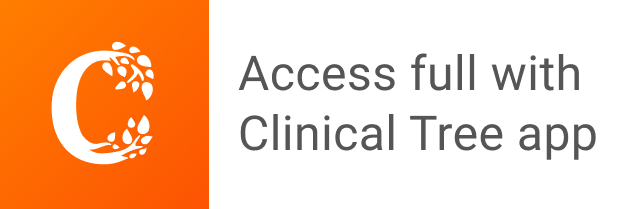