Genetic Diseases Associated with Epilepsy
Joshua L. Bonkowsky
Martha A. Nance
W. Allen Hauser
V. Elving Anderson
Introduction
Genetics has become increasingly important for understanding epilepsy. Since the advent of molecular genetic technology in the 1980s, it has been possible to categorize and diagnose many neurologic diseases that were previously poorly defined. A number of specific “epilepsy genes” have been identified and are discussed in Chapter 18. However, there are also many genetic disorders that feature epilepsy as a common or prominent symptom. In this chapter we review genetic disorders associated with an increased risk for epilepsy.
Patients having genetic or chromosomal syndromes associated with epilepsy account for 2% to 3% of all cases of epilepsy; in fact, these patients are more likely to be seen first by a pediatrician, family practitioner, or geneticist for diagnosis and treatment of the genetic syndrome. What, then, is the benefit of approaching the epilepsies using a framework of genetic classification of disease?
First, for the clinician, knowing which genetic disorders have a high risk for epilepsy can aid in differential diagnosis. The background population rate of epilepsy is 2% to 4%, whereas in some disorders, epilepsy can be present in almost 100% of patients, and the type of epilepsy can be characteristic of the disorder.
Second, investigation of the disorders that are associated with epilepsy is providing insight into the causes of epilepsy. Understanding that epilepsy is not a general consequence of all chromosomal aberrations but is present in specific chromosomal disorders, for example, has led to a search for epilepsy-related genes at specific chromosomal sites.
Third, by studying the effects of specific gene mutations on intracellular and cell membrane function, by creating animal models of human epilepsy disorders, and by studying in detail the cellular effects of human mutations, the mechanisms of epileptogenesis will be better understood. For most of the conditions described below, the actual mechanism of epileptogenesis is at present poorly understood.
Finally, for the patients and their families, diagnosis of their disorder provides the opportunity for treatment, for genetic counseling, and for tailored treatment of their seizures as well as for health maintenance issues that may arise later in the course of their disease.
Basic Genetic Concepts
Most of the conditions in this chapter involve mendelian (single gene locus) inheritance.71 Because of the rapidly changing knowledge about these disorders, we commonly use two searchable Web-based sites to help with our diagnosis and testing: GeneTests (www.genetests.org) and OMIM (Online Mendelian Inheritance in Man: www.ncbi.nlm.nih.gov/entrez/query.fcgi?db = OMIM). These sites are funded by the National Institutes of Health (NIH), and include clinical and molecular information about genetic disorders, references, and clinical laboratories that test for the different disorders.
Testing (and thus the diagnosis) of genetic disorders associated with epilepsy continues to provide challenges to the clinician. Further, the technology and means of testing is in constant flux. Again, GeneTests is an invaluable resource for finding laboratories that provide clinical testing. Unfortunately, in many instances our knowledge of the diseases has outstripped the ability of laboratories to provide clinical testing. In many diseases any one test is not 100% sensitive, so if the clinical suspicion is high enough, further testing may be warranted.
All testing should be preceded by adequate counseling about the purpose and possible outcomes of the testing and potential choices that might arise (see Chapter 19). The clinical complexity and overlap in phenotypes of these disorders, the implications for genetic testing of other family members, and ramifications on insurance coverage mandate responsible clinical judgment.
A basic grasp of genetic concepts is important for understanding the disorders in this chapter. We have grouped these topics into four areas: Genetic transmission, gene expression, genomic integrity, and genetic heterogeneity.
Genetic Transmission
Four major modes of inheritance have been characterized in detail: Autosomal dominant, autosomal recessive, X-linked recessive, and mitochondrial.
Autosomal dominant traits can be expressed when only one of the paired alleles (genes) at a given locus is of a mutant form. However, apparent “skips” in a pedigree may occur as the result of incomplete penetrance. Incomplete penetrance is the presence in an individual of a dominant disease gene without full expression of the mutant phenotype. Sometimes, however, careful examination of individuals carrying a dominant disease gene reveals minimal signs of the condition, indicating variable expression of disease symptoms.
For an autosomal recessive trait to be expressed, both alleles must be of a mutant form (although not necessarily with the same mutation). Both parents of an affected individual are heterozygous disease gene carriers, and careful examination or laboratory evaluation may sometimes reveal heterozygous effects. Ordinarily, however, carriers of a single recessive disease gene are clinically asymptomatic. Because autosomal recessive alleles are generally rare, a detailed family history should be taken to search for any consanguinity.
The hallmark of X-linked diseases is the absence of male-to-male transmission of the genetic trait, since males pass on a Y chromosome, not an X chromosome, to their sons. Thus, the condition usually is found only in male subjects who are in turn related through female carriers. Some X-linked disorders (such as incontinentia pigmenti) are lethal in affected males,
so that only female carriers of the abnormal gene survive fetal life.
so that only female carriers of the abnormal gene survive fetal life.
Mitochondrial mutations follow a maternal inheritance pattern. In this situation, the frequency of a trait is the same in male and female subjects, but the condition is always transmitted from an affected mother to most of her children, never from an affected father. A human egg contains several hundred thousand copies of mitochondrial DNA (mtDNA), compared with only a few in sperm. Theoretically, all children of an affected mother should be affected. The expression of a mitochondrial mutation, however, depends on the relative proportion of mutant and wild-type mtDNAs in a given cell (termed heteroplasmy), and this proportion can shift during cell division. Because of a “threshold effect,” tissues with a low proportion of mutant mtDNAs may not show an effect, whereas tissues that have reached the threshold begin to show effects of mitochondrial dysfunction. Organs that have a high rate of energy consumption, such as brain, heart, and muscle, are most often affected by mitochondrial disorders. It must also be noted that some mitochondrial disorders result from mutations in nuclear genes; these defects are inherited in a regular mendelian pattern.
Anticipation is the tendency for affected individuals in successive generations to present with earlier and/or more severe symptoms. This occurs in some genetic disorders, especially those associated with trinucleotide repeat expansions. Certain disorders have a predilection for repeat instability when passed on via either the paternal or maternal germ-line.
Mosaicism, or the presence of more than one genotype in the body, must also be considered in situations when multiple siblings have a disorder despite the absence of any family history of the disorder. In this situation, the parent is phenotypically normal because most of the somatic cells of the body carry the wild-type allele, but the germ cells carry the mutant allele, which is thus passed on to all the offspring.
Polygenic disorders remain a major frontier in our understanding of genetic mechanisms of disease. These disorders are common (e.g., idiopathic epilepsy) and show complex inheritance, involving multiple (possibly interacting) genes, with or without additional environmental influences. Identifying the causative genes is challenging.51
Gene Expression
The pathways from genes (the genotype) to physical or clinical traits (the phenotype) are extraordinarily complex. The molecular and developmental events that control phenotypic expression are numerous. They include DNA regulatory elements that control location, levels, rate, and timing of gene expression73; alternative splicing of the primary mRNA transcript to produce different mRNAs; imprinting, or the differential expression of a gene depending on which parent contributed the gene; regulation of mRNA expression by small RNA molecules107; and combinatorial protein and enzymatic complexes.
Genomic Integrity
Deletion or duplication of DNA segments on a chromosome can produce significant clinical effects. Larger deletions, duplications, or translocations can disrupt many genes and produce severe symptoms, including mental retardation and growth failure. Microdeletions, which may not be detectable by standard cytogenetic analysis, can produce contiguous gene syndromes that involve the loss of several neighboring genes and can lead to unique phenotypes depending upon the specific genes deleted.
Recently, a series of inherited disorders has been shown to involve the expansion of DNA trinucleotide repeat sequences. These include myotonic dystrophy, fragile X syndrome, Huntington disease, and some of the spinocerebellar ataxias. Normal individuals show variation in the number of repeats, but above a certain threshold the repeat length becomes unstable, with a tendency for further increase in number of repeats in later generations. Higher repeat numbers are associated with an earlier onset of the condition, greater severity of symptoms, or both—a phenomenon known as anticipation. The mechanisms by which trinucleotide repeat expansions cause disease remain unknown.
Genetic Heterogeneity
Genetic heterogeneity (or locus heterogeneity) is the phenomenon whereby a single clinical phenotype can result from mutations in different genes. This possibility must be considered in the genetic study of any disorder, as it may affect both diagnosis and treatment and also causes problems in gene mapping studies. Thus, the epilepsies are a limited group of phenotypes that may be caused by a number of different genes. For example, seizures can occur from changes in neuronal excitability, neuronal inhibition, or control of the spread of a seizure state, each of which is regulated by many different genes.
From a diagnostic perspective, it is not sufficient to classify epilepsy simply on the basis of the clinical or electrographic appearance of the seizures; some consideration of the underlying cause must be included. To say that a patient has mental retardation and epilepsy could miss the point that both conditions are manifestations of an underlying cause, for example, Angelman syndrome. On the other hand, a purely genetic classification is not satisfactory either, and lacks the clinical and treatment paradigms. Godfrey28 emphasized the continuing interdependence between clinicians and molecular scientists in resolving this problem:
“Do we begin to classify disorders on the basis of molecular lesions or on the basis of clinical criteria? Neither alone seems satisfactory any more. Therefore, we will use both, singly and in combination, but heterogeneity will continue to pose dilemmas in our practices and laboratories.”
Table 1 Risk for Epilepsy, and Other Seizure Associations, in Genetic Disorders | |||||||||
---|---|---|---|---|---|---|---|---|---|
|
Genetic Disorders Associated with Epilepsy
Table 1 summarizes the genetic disorders discussed in this chapter. It lists the disease name, its associated gene (if known), and seizure frequency. We have organized the genetic disorders in the following broad categories:
Chromosome disorders
Contiguous gene disorders
Metabolic disorders
Genetic syndromes; which are further subdivided as follows:
Short stature
Early overgrowth
Skeletal dysplasias
Facial defects
Connective tissue
Neurocutaneous
Ectodermal and mesodermal dysplasias
Disorders of brain development
Neurodegenerative disorders
Chromosome Disorders
The chromosome disorders represent a wide array of possible disorders caused by additions, deletions, or rearrangements of chromosomal material (see Chapter 261). Classical cytogenetic methods are now complemented by more sensitive methods such as high-resolution chromosome banding, fluorescent in situ hybridization (FISH), and the polymerase chain reaction (PCR). Small deletions or duplications are now detectable, and the contribution of an individual gene to the overall phenotype of a chromosome disorder can often be determined.
Six percent of patients with epilepsy and intellectual impairment have chromosomal abnormalities, while 50% of patients with epilepsy and multiple congenital abnormalities have chromosomal abnormalities, as noted by Singh et al.105 in their review of the frequency and types of epilepsies resulting from disorders of each of the chromosomes (see also the review by Battaglia and Guerrini4).
Epilepsy occurs in 1% to 10% of patients with trisomy 21 syndrome (Down syndrome), and electroencephalographic (EEG) abnormalities are present in more than 20%.21,104,118 EEG abnormalities ranged from slowing, asymmetry, and asynchrony to diffuse or focal epileptiform activity.21 Seizures most often begin early in childhood, and can be of different types; infantile spasms occur occasionally (0.5% to 2% of patients), while myoclonic seizures are distinctly uncommon.89,104,123
Trisomy 13 syndrome is commonly accompanied by severe developmental retardation, microcephaly, and severe craniofacial dysgenesis, including holoprosencephaly. Seizures occur in 25% of patients and apneic spells in 58%.33
Trisomy 18 syndrome, the third common autosomal trisomy, is characterized by poor somatic growth and hypoplasia and dysplasia of many internal organs, including the brain. Gross malformations of the brain range from heterotopias to holoprosencephaly, which may be associated with apneic episodes and seizures.33 Others report a much lower incidence of seizures in trisomy 18 patients than in trisomy 13.125
More than 50% of patients with Wolf-Hirschhorn syndrome (del4p16) have seizures, which can be difficult to control. Characteristic EEG and seizure patterns (including infantile spasms) are associated with this syndrome.3 The incidence of seizures may depend on the exact site of the deletion, as the “critical region” is still being delineated. In contrast, patients with cri-du-chat syndrome (del[5p]) are microcephalic but generally do not have severe seizures.
The fragile X syndrome is included here as a chromosome disorder for historical reasons. The first diagnostic test for this disorder was cytogenetic, based on the presence of a visible disruption in the X chromosome (the “fragile X”) when cells of affected individuals were grown in a culture medium low in folate. Fragile X syndrome is now known to be a trinucleotide repeat disease caused by the expansion within the FMR-1 gene of a trinucleotide that is repeated in tandem only a few times in normal individuals. Fragile X syndrome is responsible for about 2% to 5% of all cases of mental retardation in male individuals, and also has been associated with mental retardation in females, although usually less severe than in their
male relatives. In large series, about 20% of males and 5% of females with fragile X syndrome have clinically apparent seizures, and about 50% have abnormal EEGs.6,121 No patient had infantile spasms. EEG abnormalities included nonspecific slowing as well as epileptiform or paroxysmal discharges; other authors have reported a high incidence of centrotemporal (rolandic) spike discharges in FMR-1 mutation carriers in the second half of the first decade of life, both with and without clinical seizures. Seizures and EEG abnormalities in individuals with fragile X syndrome tend to improve with age.
male relatives. In large series, about 20% of males and 5% of females with fragile X syndrome have clinically apparent seizures, and about 50% have abnormal EEGs.6,121 No patient had infantile spasms. EEG abnormalities included nonspecific slowing as well as epileptiform or paroxysmal discharges; other authors have reported a high incidence of centrotemporal (rolandic) spike discharges in FMR-1 mutation carriers in the second half of the first decade of life, both with and without clinical seizures. Seizures and EEG abnormalities in individuals with fragile X syndrome tend to improve with age.
Other less common chromosome deletion and translocation syndromes are associated with the presence of epilepsy. Seizures were present in a series of over 400 different chromosome imbalances105; eight disorders were highly associated with epilepsy: Wolf-Hirschhorn (4p-) syndrome, Miller-Dieker syndrome (del 17p13.3), Angelman syndrome (del 15q11-q13), the inversion duplication 15 syndrome, terminal deletions of chromosome 1q and 1p, and ring chromosomes 14 and 20. Notably, epilepsy is not a prominent feature of the sex chromosome aneuploidies, such as Turner syndrome or Klinefelter syndrome. Subtelomeric deletions at the ends of chromosomes have been reported to be a significant cause of mental retardation,61 but seizures appear to only be an occasional association.
Contiguous Gene Disorders
Three contiguous gene disorders associated with seizures are discussed below: Angelman syndrome, Prader-Willi syndrome, and Velocardiofacial syndrome (Miller-Dieker syndrome is discussed later in this chapter). Other contiguous gene disorders not associated with an increased risk of seizures include Williams syndrome, WAGR (Wilms tumor, aniridia, genitourinary anomalies, and mental retardation) syndrome, Potocki-Shaffer syndrome, and Smith-Magenis syndrome.
Angelman syndrome was first identified cytogenetically as involving loss of the maternal chromosome region 15q11-13. Affected patients have severe mental retardation, epilepsy, ataxic jerky movements, inappropriate laughter, and absence of speech. UBE3A, a ubiquitin-protein ligase, is the minimal gene mutation responsible for the core phenotype, but the phenotypic presentation depends on the type of mutation and which associated genes are involved.60,70
Seizures with onset at <3 years of age and EEG abnormalities are findings in more than 80% of patients, and are part of the diagnostic criteria for Angelman syndrome.120 Abnormal EEGs are commonly present in infancy and may be the first diagnostic sign.8,24 EEG abnormalities in infants often include irregular, generalized, high-voltage sharp-wave and spike-wave activity. Although only 30% of patients have seizures in the first 2 years of life, by 3 years of age 85% will have developed seizures, most often of the absence, myoclonic, or generalized tonic–clonic types. However, some of the abnormal movements in Angelman syndrome are cortically based myoclonus, responsive to GABAergic (γ-aminobutyric acid) drugs.
Prader-Willi syndrome also involves chromosome region 15q11-13, but in contrast to Angelman syndrome, results from loss of the paternally derived chromosome. Patients with Prader-Willi syndrome have infantile hypotonia, short stature, small hands and feet, hypogonadism, and mental retardation, and as children develop hyperphagia and obesity.37 Seizures are present in 15% to 20% of patients, but in contrast to Angelman syndrome, the seizures are not severe, chronic, or therapeutically challenging.9 Molecular diagnosis is based on abnormal methylation studies, which detect 99% of cases.13
Velocardiofacial syndrome, a contiguous gene deletion involving chromosome region 22q11.2, is characterized by congenital heart disease, palatal abnormalities, characteristic facial features, learning difficulties, and immune deficiency. Former names for velocardiofacial syndrome are DiGeorge syndrome and Shprintzen syndrome. Seven percent of patients with velocardiofacial syndrome develop seizures.57 In addition, infants with velocardiofacial syndrome sometimes present with seizures secondary to hypocalcemia because of parathyroid deficiency.
Metabolic Disorders
There are several general mechanisms by which metabolic disorders can result in seizures (see also Chapters 261 and 262). First, any disorder that alters the cellular metabolic environment (such as hyperammonemia) can provoke seizures. Second, some metabolic disorders can lead to secondary structural abnormalities in the brain. For instance, cortical infarctions can develop in patients with MELAS (mitochondrial encephalomyopathy with lactic acidosis and strokelike episodes) that can act as epileptogenic foci even in the absence of acute systemic metabolic disturbance. Finally, a tendency to epilepsy may be intrinsic to the metabolic disturbance or disease itself, if the metabolic pathway is necessary for the function of neurons and/or glia. The treatment of seizures in patients with metabolic disorders must include treatment of the metabolic derangement; seizures are likely to persist despite anticonvulsant therapy if an untreated metabolic abnormality is present, and anticonvulsant therapy may not be necessary if the metabolic derangement is brought under control.
Wolf et al.124 reviewed the main characteristics of the epilepsies that are found in the inborn errors of metabolism and outlined a general approach to diagnosis and treatment. For more detailed information see also The Molecular and Metabolic Basis of Inherited Disease,103 Diagnostic Recognition of Genetic Disease,81 and Atlas of Metabolic Diseases.80
In patients with disorders of carbohydrate metabolism and glycogen storage diseases, seizures occur in association with hypoglycemia or during times of metabolic stress. Accordingly, seizures occur in 25% to 40% of patients with glycogen storage disease types I and III and rarely in other types, in which systemic hypoglycemia is uncommon. Patients with d-glyceric acidemia and galactosemia have myoclonic seizures, and abnormal waking EEGs are seen in more than 50% of children with galactosemia.
Niemann-Pick disease, type C (NPC) is a lipid storage disease with a variable age of onset, typically presenting with ataxia, vertical supranuclear gaze palsy, dementia, and, in infants, hypotonia and organomegaly.85 Inheritance is autosomal recessive; most cases are caused by mutations in NPC1, and diagnosis is based on impaired cholesterol esterification in cultured fibroblasts that show positive filipin staining. About one third of patients with NPC have seizures, which are often resistant to treatment. Ironically, seizures tend to decrease with prolonged survival, possibly due to continued neuron loss. Gelastic cataplexy, the sudden loss of muscle tone from humorous stimuli, is present in about 20% of children with NPC.56,88
A number of the disorders of amino acid metabolism (including the urea cycle disorders) feature seizures or epilepsy. The urea cycle defects are especially marked by significantly elevated levels of ammonia; about 50% of neonates with elevated ammonia will have seizures, along with cerebral edema.110 The use of valproic acid is contraindicated in these disorders because it can raise ammonia levels. Seizures are also prominent in HHH (hyperammonemia, hyperornithinemia, hypercitrullinemia) syndrome, nonketotic hyperglycinemia, and homocystinuria.
HHH syndrome, like other disorders of the urea cycle, may present at any time from infancy to adulthood with lethargy, episodes of vomiting or neurologic dysfunction, seizures, and
coma. It is somewhat more likely to appear later in life than other disorders of the urea cycle, which usually develop in infancy or childhood.
coma. It is somewhat more likely to appear later in life than other disorders of the urea cycle, which usually develop in infancy or childhood.
Nonketotic hyperglycinemia (NKH) presents in infancy with intractable generalized or myoclonic seizures, hypotonia, apnea, and high levels of glycine in the blood and cerebrospinal fluid (CSF). NKH has autosomal recessive inheritance, and is caused by mutations in the glycine cleavage system. The EEG most commonly shows a burst-suppression pattern, but hypsarrhythmia can also be seen. NKH should be considered in the differential diagnosis of a patient with infantile spasms. Most patients die before 1 year of age, although affected males have a longer survival rate and better neurologic function.48 Diagnosis can be made by detecting elevated levels of glycine in the serum, but in some cases CSF amino acid analysis is necessary, as glycine levels may only be elevated in the CSF and not in the serum. Brain magnetic resonance imaging (MRI) can be useful for diagnosis as well, as reported abnormalities include ventriculomegaly, absent corpus callosum, posterior fossa cysts, delayed myelination, areas of diffusion-restriction, and an elevated glycine peak on magnetic resonance spectroscopy (MRS).59 Treatment with standard anticonvulsants may not be successful, and treatments directed toward lowering glycine levels have met with only mild success.
Homocystinuria is a relatively common metabolic disorder. The frequency of epilepsy in homocystinuria has been estimated at 21%, with the most common seizure type being generalized tonic–clonic seizures.74 These seizures are not associated with any identifiable metabolic “crisis,” and epileptiform EEG findings are common both in patients with seizures and in patients who are clinically asymptomatic.17
Pyridoxine-dependent seizures (PDS) occur within the first hours or days of life and respond dramatically to treatment with vitamin B6. PDS has autosomal recessive inheritance, and several different gene loci have been implicated.5 Any infant presenting with idiopathic seizures in the first week of life warrants a trial of pyridoxine, as appropriate treatment can lead to a seizure-free, neurologically normal outcome.115
Seizures commonly accompany the metabolic crises of not only the urea cycle disorders, but also of the organic acidurias, such as propionic acidemia, isovaleric acidemia, biotinidase deficiency, and glutaric aciduria, type I. The most common seizure types are generalized myoclonic or infantile spasms. In general, seizures are more likely to be a presenting feature in infants or young children with these disorders than in children with variants of later onset. Biotinidase deficiency can present with ataxia, developmental delay, seizures, and an eczematous rash. Seizures were the presenting feature in 38% of patients, and 55% of patients had seizures.100 Glutaric aciduria, type I, is an autosomal recessive disorder presenting with macrocephaly, a progressive movement disorder that can be misdiagnosed as cerebral palsy, and progressive neurologic symptoms that may acutely worsen during periods of illness.47 MRI may show frontotemporal atrophy and enlarged CSF spaces in the sylvian fissures (“bat-wing” appearance)80 (see also Chapter 262). Seizures more commonly accompany the acute decompensatory episodes.
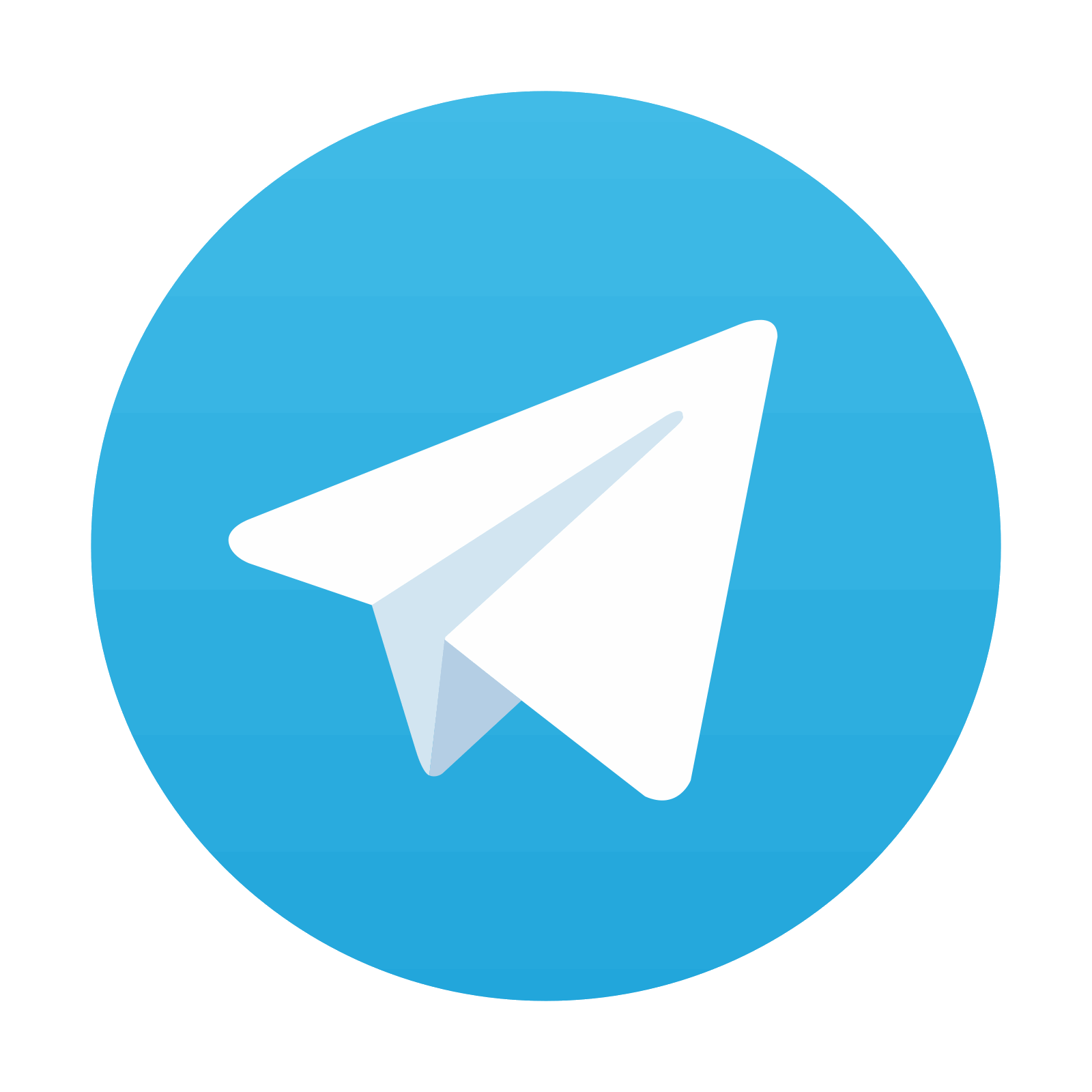
Stay updated, free articles. Join our Telegram channel
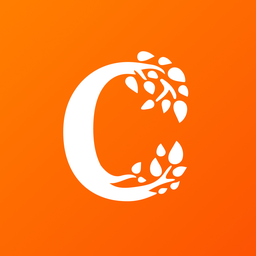
Full access? Get Clinical Tree
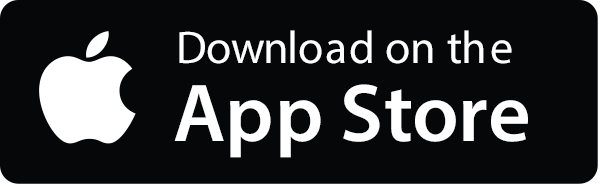
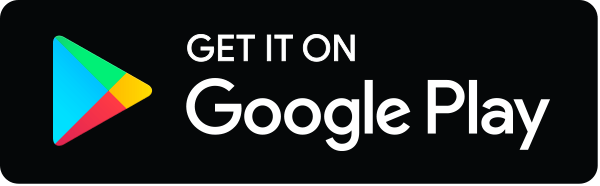