Keywords
Genetic testing, genetic, genomic, mutation, chromosome microarray, DNA sequencing, whole exome sequencing, muscular dystrophy, Duchenne muscular dystrophy
Introduction
Many neuromuscular disorders in children are single gene disorders, and therefore genetics plays a primary role in the diagnosis of many neuromuscular conditions. Establishing a definitive molecular genetic diagnosis provides clarity about prognosis; suggests possible therapeutic strategies; allows patients, families, and providers to access appropriate clinical trials, research studies, centers of excellence, and family support organizations; and provides parents and other family members with critical information to accurately determine risk of recurrence and reproductive options to increase the probability of having a healthy child in the future. Increasingly, the exact type of mutation may also suggest possible therapeutic strategies, and it will become increasingly important to identify the exact mutation(s) in clinically affected individuals even when the clinical diagnosis is not in question. Molecular genetic diagnostics have been revolutionized by the decreased cost of sequencing and elimination of patents on genes, and improvements in genetic diagnostics should allow for increasingly efficient, expedient, and cost-effective methods to establish a diagnosis so patients can move beyond diagnosis and on to treatment.
This chapter will briefly review the basic principles of genetics and the principles of molecular genetics, and then focus largely on the clinical application of genetics to provide new approaches to diagnosis, counseling, and treatment. Given the uneven access to clinical genetics services outside major academic medical centers, the burden of the increased demand of genetic evaluation will fall largely on the neurologist who diagnoses and manages the neuromuscular patient. Thus, neurologists need to be conversant with the technologies and principles of genetic evaluation, testing, and counseling.
Patterns of Genetic Transmission
The human genome consists of approximately 3 billion base pairs of DNA packaged into 23 pairs of chromosomes. The 23 pairs of nuclear chromosomes consist of 22 non-sex chromosomes, called autosomes, and two sex chromosomes, the X and the Y. In addition, there are approximately 16,500 base pairs of DNA located on circular double-stranded molecules within mitochondria, referred to as the mitochondrial genome. Recessively inherited diseases require mutations in both copies of the gene whereas dominantly inherited conditions require mutations in just one of the two copies of the gene. X-linked conditions such as Duchenne muscular dystrophy more commonly manifest in males since males have only a single X chromosome and lack the second copy of most of the genes on the X chromosome as a backup if there is a mutation in an X-linked gene. Mitochondria are exclusively maternally inherited, so mutations of the mitochondrial genome are maternally inherited.
Pedigree Analysis and Risk Assessment
Taking a three-generation family history should be a standard part of any initial clinical evaluation. This is most efficiently performed by having patients or their parents complete a brief tabular questionnaire about the family history at home or in the waiting room so the provider can review the pertinent positives while taking the medical history. Some practices have a patient portal to facilitate collection of family history data. A sample family history screening tool is shown in Figure 2.1 , and an online tool is also available. It is important to remember to ask about deceased relatives, since some people forget to mention them when a family history is taken. Individuals who have had multiple partners should be noted, and full siblings should be distinguished from half siblings as this may provide important clues to a dominantly inherited disorder such as myotonic dystrophy. Consanguinity can be screened by asking if the child’s parents are related by blood, which is common in some cultures. The ethnic background of both sides of the family should be noted since hereditary diseases vary in prevalence by ethnicity.

Assessment of recurrence risk is extremely important for many families who are considering having more children and is often difficult in small families. Genetic testing can be helpful to confirm the diagnosis, establish a precise risk of recurrence, and provide the family with options for future family planning. For an autosomal recessive disorder, parents are usually both asymptomatic carriers for the condition, and neither parental side may report a prior family history of the condition. If the affected child has no siblings, or is a member of a small family with no affected siblings, parents will often dismiss the possibility of a genetic etiology because they have never observed this condition within their family. However, parents who are asymptomatic carriers of an autosomal recessive condition have a 25% risk of recurrence with each pregnancy.
Increasingly, couples considering having children are offered carrier screening for a variety of autosomal recessive conditions, and some obstetricians include carrier screening for spinal muscular atrophy (SMA) in that panel. However, it is important to remember that screening tests are usually not comprehensive and are not 100% sensitive; therefore, even if parents have had normal carrier screening for a condition within the child’s differential, the child could still have the disease due to a mutation not included within the carrier screening panel or due to technical limitations of the carrier screening test.
Autosomal recessive conditions are more frequent when there is a family history of consanguinity, but consanguinity does not guarantee that the condition will be recessive. When the child has two copies of the same mutation, the child is homozygous for the mutation. When the child has two different mutations, the child is a compound heterozygote. Occasionally, a child will be found to carry more than two genetic variants in a gene, and the inheritance of the variants can be most readily determined by testing the parents to determine which variants are in cis (contained on the same copy of the gene and inherited together from one parent) and which are in trans (contained on separate copies of the gene and inherited from each parent separately).
Individuals with an autosomal dominant disorder have a 50% chance of passing the mutation on to any offspring, as is the case for conditions such as myotonic dystrophy or Charcot-Marie-Tooth type IA. In children, a significant percentage of severe and lethal diseases in childhood that are dominantly inherited are due to de novo or new mutations in the child. Although genetic testing from blood in the parents is normal, there is a small chance that one of the parents is a gonadal mosaic and has multiple germ cells carrying the mutation even though the blood cells do not carry the mutation. A 1% risk of recurrence is given to such parents who have a child with a de novo mutation. It is important to note that if a child carries a de novo mutation for a dominant condition, the mutation becomes a germline mutation in the child and the risk of recurrence for that child’s children will be 50%.
For X-linked disorders, the recurrence risk depends solely upon the mother’s genetic status and does not rely upon the father’s genetic status. The lack of male-to-male transmission of the genetic disease is the hallmark of X linkage. If the mother is a carrier for the genetic mutation, there is a 50% risk that her sons (regardless of her partner) will inherit the condition and will be symptomatic while 50% of her daughters will be carriers and unlikely to have classical symptoms for most disorders due to the random process of lyonization or X inactivation in the relevant tissue. However, milder manifestations in female carriers are more common. As an example, some women who are carriers for Duchenne muscular dystrophy will have a mild elevation of CPK and a small percentage will develop signs and symptoms of cardiomyopathy, although most will not develop clinically significant muscular dystrophy. For some X-linked disorders, again using Duchenne muscular dystrophy as an example, there is a high frequency of spontaneous mutations. Women who have sons with spontaneous mutations have a 1% risk of recurrence in future pregnancies due to the small chance of gonadal mosaicism as described previously.
Risk assessment in mitochondrial disorders is more difficult because the genes responsible for mitochondrial function can be encoded in the nuclear or mitochondrial genes, so that inheritance can be either autosomal, rarely X linked, or maternal. The majority of proteins involved in oxidative phosphorylation in the mitochondria are encoded in the nucleus, but there are 13 proteins involved in oxidative phosphorylation, as well as a full set of transfer and ribosomal RNAs encoded in the mitochondrial genome (see Chapter 41 ). Because the brain and skeletal muscle have high energetic demands, clinical manifestations of mitochondrial disorders are often neuromuscular. Nuclear encoded genes lead to straightforward risk of recurrence, but mitochondrial encoded genes are difficult to predict in the next generation due to heteroplasmy; that is, the mitochondria are, in fact, a population of organelles with only a subset of the organelles carrying the mutation ( Figure 2.2 ). Only the maternal contribution is relevant for disorders encoded within the mitochondria since all mitochondria in the embryo and fetus are derived from the egg. From one egg to the next, the percentage of mitochondria with the mutation varies and is not predictable, and within the developing fetus as cells divide by mitosis the distribution of mutant mitochondria between tissues can vary and give rise to significant heterogeneity in severity and affected organs. Thus, prenatal diagnosis for mutations encoded within the mitochondria is imprecise and can be inaccurate.

For all modes of inheritance, the genetic information for a gene is referred to as the genotype; the manifestations of that gene are referred to as the phenotype, and in medicine this largely refers to the clinically observable signs and symptoms of disease. For most pediatric neuromuscular conditions, the penetrance or the probability of expressing a manifestation of a genetic mutation is usually high and often 100%. However, in many cases, manifestations may not be present at birth, so penetrance can be age dependent. In genetic conditions, the phenotypic manifestations may differ from person to person, a phenomenon referred to as variable expressivity .
Chromosomes and Chromosomal Abnormalities
Aside from mutations in single genes, genetic abnormalities may result from contiguous gene defects of entire chromosomes or parts of chromosomes. Children can be born with extra or missing chromosomes (called aneuploidy), often associated with neonatal hypotonia. With the exception of the X and Y chromosomes, having a missing chromosome tends to be lethal during embryonic or fetal development. Trisomy is lethal for most autosomes during the prenatal period but is compatible with life for chromosomes 13, 18, and 21, although each of these is associated with moderate to severe intellectual disability. Chromosome rearrangements may occur within a chromosome, such as deletion, duplication, or inversion, or may involve exchange of genetic material between a pair of chromosomes, referred to as translocation. Translocations are balanced when no genetic material is gained or lost overall. Balanced translocations are less likely to result in disease compared to unbalanced translocations, but disruption of a gene at the translocation breakpoint can lead to clinical manifestations that are often quite specific and well defined. Deletions are generally more severe than duplications, and in general the larger the deletion or duplication and the greater the number of genes involved, the more severe are the clinical manifestations. New, extremely sensitive technologies called chromosome microarrays are now available to detect segmental aneuploidies or copy number variants associated with microdeletions or microduplications.
Genetic Imprinting
For a small number of genes, the parent of origin is paramount because only one copy of the gene is expressed. Whether the maternal or paternal copy of the gene is expressed is consistent for each imprinted gene, but some genes along a chromosome segment will be maternally expressed and paternally imprinted (silenced) while other genes in close proximity on the same chromosome will be paternally expressed and maternally imprinted (silenced). An example of imprinting is the Prader-Willi syndrome/Angelman syndrome locus on chromosome 15. Prader-Willi syndrome often presents as neonatal hypotonia and is due to defects in the paternal copy of the SNRPN locus on 15p, including deletions of the paternal locus, two maternal copies of chromosome 15 (uniparental disomy for chromosome 15), or rarely mutations in the imprinting locus. Angelman syndrome is due to defects in the maternal copy of the nearby UBE3A gene on chromosome 15p, including deletions of the maternal gene, two paternal copies of chromosome 15, mutations in UBE3A , or rarely mutations in the imprinting locus. The imprint is erased and reset at each generation. Imprinting disorders, when inherited, can thus have a peculiar pattern of inheritance because of appearing to skip generations. However, most imprinted disorders in pediatric neuromuscular conditions arise as de novo mutations, and there is rarely a family history of the disorder.
Molecular Genetics
Understanding the basic anatomy of a gene is necessary to appreciate the nature and consequences of genetic mutations and to understand the limitations of genetic tests. A prototypical gene is shown in Figure 2.3 . Transcription begins with the binding of RNA polymerase to the promoter, and the full length of the gene is then copied into RNA. There may be enhancers of gene expression located at some distance from the promoter located upstream or downstream of the gene, or embedded in the middle of the gene in an intron. The gene is composed of exons and introns. After transcription is complete, the introns are removed by splicing to produce the messenger RNA (mRNA), which is exported from the nucleus and translated into a protein in the cytoplasm. The sequences at the junction of the intron and exon are critical for correct splicing, and consensus sequences mark the splice donor and splice acceptor sites. Splice acceptors always end in an AT sequence, preceded by a “consensus sequence,” and donors begin with GC, followed by a conserved consensus sequence. Mutations in these sequences at the splice junctions can result in aberrant splicing, leading to an abnormal mRNA and abnormal protein product. Mutations can also occur deep within introns or as synonymous changes (which do not change the amino acid encoded by the codon) within the exons leading to alternative splice sites that can also lead to aberrant splicing.

Splice mutations at the splice junction are readily detected by most molecular genetic methods used in genetic testing. However, with the exception of the small number of genetic tests that are preformed directly on the mRNA, mutations leading to aberrant splicing outside the splice junction can easily be missed because they may not be included within the target for sequencing. Mutations in enhancers are also frequently missed by most genetic tests because they have not been well defined and are not included in the sequence analyzed in most genetic tests.
Gene Mutations and Modes of Inheritance
The mechanism by which a sequence change affects the protein and its function in part determines the mode of inheritance. Most recessive disorders are due to loss of function mutations. In general, the more severe the loss of function, the more severe the clinical manifestations, or the earlier the age of onset for recessive diseases. Certain mutations lead to complete gene disruptions and include whole gene deletions, multiple exon deletions/duplications, most premature termination mutations except those at the end of the protein, and most frameshift mutations except those at the end of the protein. Exon deletions/duplications are more deleterious when they also result in a change in the reading frame of the protein distal to the deletion. Splice mutations disrupt genes to a variable degree depending on how leaky the mutation is, and how much of the normal splice product is made. Promoter and enhancer mutations have variable severity. Missense mutations that result from the substitution of one amino acid for another can have a wide range of effects from severe to minimal change in function of the protein depending on how critical that amino acid is in the protein and how significant the difference is in the properties of the amino acid substitution (e.g. size, charge, ability to make disulfide bonds). Loss of function mutations are inherited in either a dominant or recessive manner depending on how much of the protein product is necessary for normal function. For many enzymes as little as 10–20% of the enzymatic activity may be compatible with a normal phenotype. Disorders encoded by such enzymes are usually inherited as autosomal recessive conditions in which carriers are asymptomatic. In general, the lower the level of enzymatic activity, the earlier the age of onset and the more severe the clinical features. For other genes, the gene product is much more tightly regulated, and loss of 50% of the normal gene product can produce significant problems. Disorders encoded by these tightly regulated genes are usually inherited as dominant conditions and include many developmental disorders in which gene expression is finely tuned to support normal organogenesis.
Missense mutations can rarely result in gain of a novel function to the protein or may result in an increase in activity or expression of the protein. Such mutations are usually inherited in a dominant manner. In addition, some loss of function mutations are within protein subunits that make up multimeric proteins. In such cases, because only one aberrant subunit can significantly impair function of the entire complex, a mutation in one allele may be amplified to affect more than 50% of the complexes and can be inherited in a dominant manner.
Triplet repeat expansions represent a unique type of mutation. Triplet repeats consist of three nucleotides such as CGG, CTG, or CAG, which are repeated multiple times. These repeats can be located in various parts of the gene, including a CGG repeat in the promoter in fragile X syndrome that interferes with the promoter of FMR, a CTG repeat in the 3′ untranslated region in myotonic dystrophy that interferes with RNA processing of many proteins, a GAA repeat within an intron in Friedreich’s ataxia which interferes with RNA splicing, or a CAG repeat within an exon encoding a polyglutamine tract in Huntington’s disease or spinocerebellar ataxias leading to accumulation of mutant protein that is toxic to the cells. All of these triplet repeats are inherited as autosomal dominant disorders except for Friedreich’s ataxia. There can be normal variation in size in the number of repeats for each of these triplet repeats, but when the repeat sizes go beyond a specific threshold that is unique for each gene, pathology ensues: the larger the repeat, the earlier the age of onset and the more severe the clinical phenotype. For some repeat disorders, there is anticipation of the disease and younger age of symptom onset with expansion of the repeat from one generation to the next (typically associated with transmission by a specific gender parent that is specific for each gene). As an example, myotonic dystrophy is associated with anticipation with maternal transmission, and a mildly affected mother can have a child with a severe form of congenital myotonic dystrophy.
Developments in Molecular Diagnostics
In the past several years, genetic diagnosis has become an important tool in confirming clinical diagnoses, providing improved prognostic information, predicting risk of disease for unaffected family members, and offering reproductive options for families to avoid transmission of heritable diseases to the next generation. The number of available clinical genetic tests has increased significantly in the last decade, and they are increasingly becoming much more comprehensive, even including genomic analyses in some cases. Previously, the heretofore relatively high cost of dideoxy sequencing had limited the potential impact of medical sequencing to diseases of small genes and diseases caused by one or two genes. The availability of next-generation sequencing methods and the elimination of patents on genes by the Supreme Court have dramatically reduced the cost of sequencing, which should continue to significantly reduce the cost of clinical genetic tests, increase the clinical utility of currently offered tests by expanding genetic coverage of currently tested genes or adding additional genes, and enable sequencing tests for diseases with extreme clinical heterogeneity such as myopathies and neuropathies.
A genetic test must meet several requirements before it is offered to the medical community. The primary requirement is that the test must have clinical utility. This means that the result should be useful to the physician in one or more of the following ways: (1) by establishing or confirming the clinical diagnosis or predicting an increased future risk of disease, (2) providing the physician with more accurate information that allows development of a plan for surveillance or response to therapy that improves clinical outcome, (3) providing prognostic information, and (4) providing the patient and his/her family with information that can be used for family planning purposes (e.g. prenatal diagnosis, preimplantation genetic diagnosis). As molecularly based intervention for specific mutation types becomes available using molecular chaperones for missense mutations or read through of premature terminations, it will be critical to understand the genetic basis for all genetics conditions to facilitate molecular therapeutics.
A genetic test should also have high analytic and clinical sensitivity. Analytic sensitivity is a measure of the proportion of tested samples that correctly give a positive result by the test method and is a measure of how well the test is technically performing in the laboratory. Clinical sensitivity measures the proportion of individuals who demonstrate a positive result using the test method as a fraction of those who have the disease. In addition, the genetic test should have high analytic and clinical specificity. Analytic specificity is a measure of the proportion of tested samples that are truly negative of all negative results by the test method. Clinical specificity measures the proportion of all unaffected individuals identified by the test method as test negative.
The development of a clinical test becomes especially complex in the case of a disorder in which a mutation in any one of several genes may underlie the disease (genetic heterogeneity). In many cases, mutations in any one of several genes can lead to similar clinical manifestations (phenocopies) that are clinically indistinguishable. Genetic testing for these diseases has proven difficult because the number of genes to be tested is often large, and may require complete analysis of as many as 10 or more genes. In addition, because mutations are “private” or unique to each family in many diseases, including those that are genetically heterogeneous, complete sequence analysis is required to ensure high clinical sensitivity. Examples of disorders for which any one of multiple genes with private mutations could underlie the phenotype in a given family include intellectual disabilities, hypotonia, Charcot-Marie-Tooth, congenital muscular dystrophies, or muscle eye brain disease.
Usually, genes that account for a large proportion of a clinical phenotype are the first to be identified and reported in the scientific literature. Heretofore, most laboratories have provided testing of only those most common genetic causes, in an effort to minimize the sequencing burden and to provide a diagnosis for the largest fraction of patients at the lowest cost for the test. However, as new genes are identified, the number of disease-causing genes for which testing is appropriate also increases. As a result, a cascade of tiered (or “reflex”) genetic tests has been offered for neuropathies such as Charcot-Marie-Tooth, starting with genes for which mutations are more common and then proceeding to the genes for which mutations account for a smaller proportion of cases. However, with the advent of next-generation sequencing, it is now possible to quickly and cost effectively perform testing for as many as 50–100 genes in a panel for a specific clinical indication. All genes are analyzed simultaneously in parallel rather than sequentially. With the advent of targeted genetic therapy based upon molecular etiology and mutation type, such as antisense oligonucleotide therapy for Duchenne muscular dystrophy to induce skipping of the targeted exon by modulating pre-mRNA splicing, restore the open reading frame of Dystrophin, and produce internally deleted, yet partially functional dystrophin protein isoforms, it has become increasingly important to be able to accurately define the specific gene and mutation causing a patient’s disorder. Thus, there is a need to offer extensive testing of as many genes as possible so that therapeutic options, as well as accurate information about recurrence risk and effective reproductive options, are available to individuals with the disease and their family members.
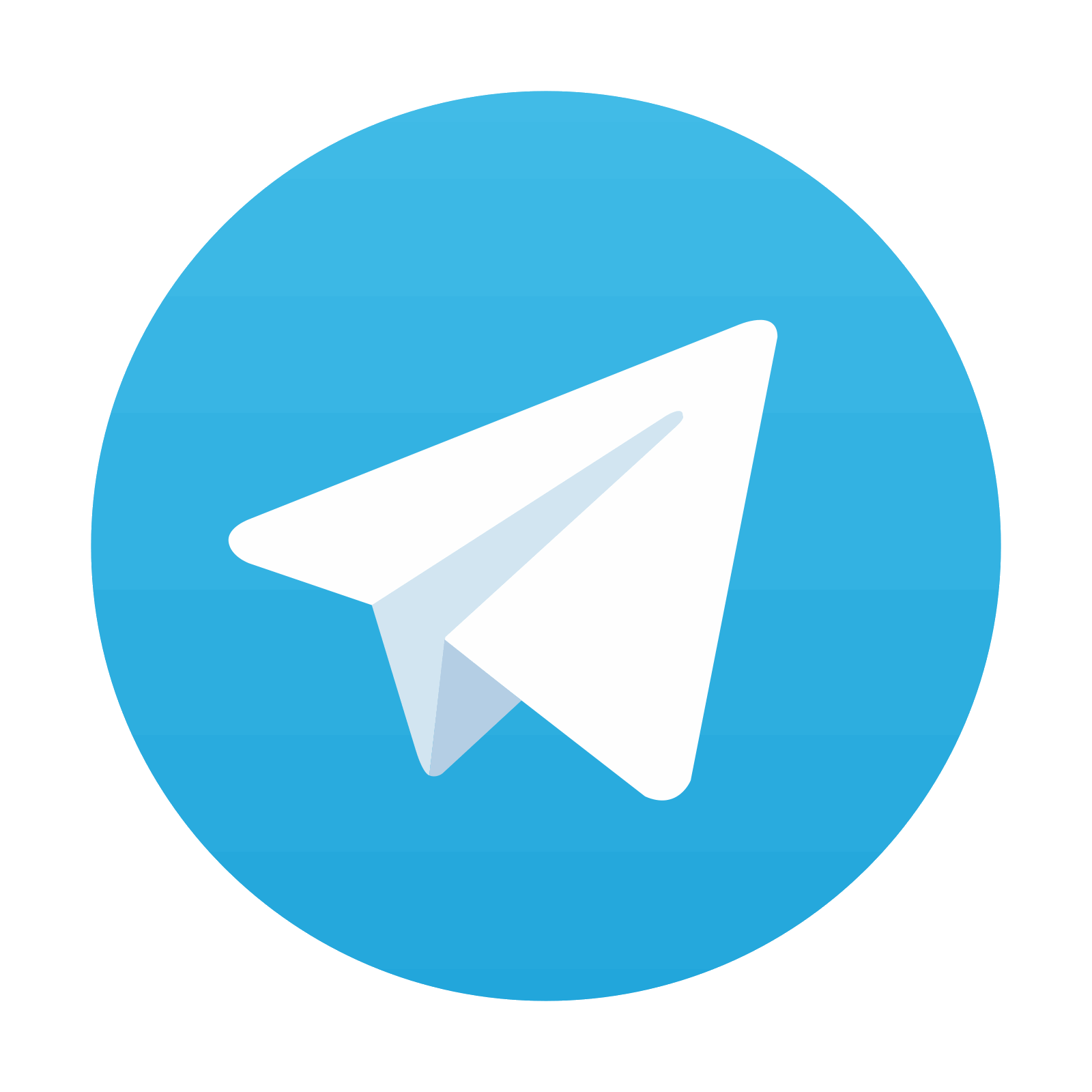
Stay updated, free articles. Join our Telegram channel
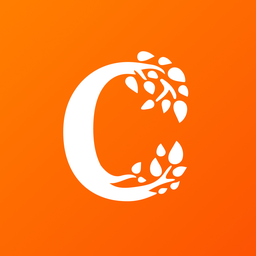
Full access? Get Clinical Tree
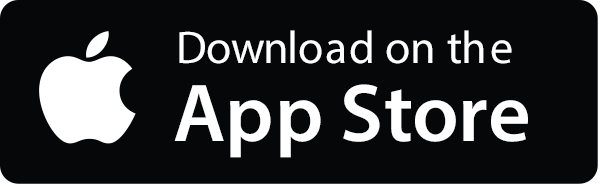
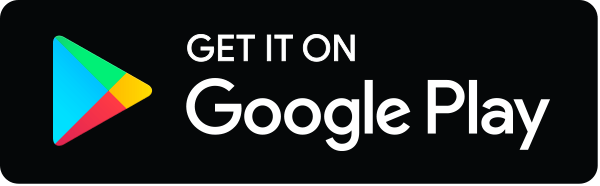