Keywords
Congenital myasthenic syndrome, neuromuscular junction, EMG, fetal akinesia syndrome, choline acetyltransferase, ColQ, β2-laminin, acetylcholine receptor, rapsyn, agrin, MuSK, Dok-7, GFPT1, DAPGT1, ALG2, ALG14, plectin
Introduction
Congenital myasthenic syndromes (CMS) are heterogeneous disorders in which the safety margin of neuromuscular transmission is compromised by one or more specific mechanisms. These syndromes are neither new nor uncommon. They were recognized by Rothbart, who in 1937 described four brothers with myasthenia younger than 2 years of age in one family, and by Sarah Bundey, who in 1972 collected 97 familial cases with onset in early childhood. After the discovery of the autoimmune origins of myasthenia gravis in the 1970s and of the Lambert-Eaton syndrome in the 1980s, it became apparent that myasthenic disorders occurring in a familial or congenital setting must have a different etiology. Since then, clinical, ultrastructural, in vitro microelectrode, and molecular genetic studies of CMS patients have revealed a phenotypically and genetically heterogeneous group of disorders. To date, no fewer than 19 CMS disease genes have been identified. Most disease proteins reside in presynaptic, synaptic basal lamina, or postsynaptic endplate (EP) compartments, but recently it has become apparent that proteins distributed in many tissues, such as those providing cytoskeletal support or subserving glycosylation of nascent peptides, are also CMS targets ( Figure 26.1 ).

Diagnosis
Generic Diagnosis
A generic diagnosis of a CMS can be made on clinical grounds from a history of fatigable weakness involving ocular, bulbar, and limb muscles since infancy or early childhood, a history of similarly affected relatives, a decremental electromyographic response, and negative tests for antibodies against the acetylcholine receptor (AChR) and the muscle specific receptor tyrosine kinase (MuSK). In some CMS, however, the onset is delayed, the electromyography (EMG) abnormalities are not present in all muscles or are present only intermittently, and the weakness has a restricted distribution. Box 26.1 lists the differential diagnosis of CMS.
Neonatal Period, Infancy, Childhood
- •
Spinal muscular atrophy
- •
Congenital myopathies (central core disease, nemaline myopathy, myotubular myopathy)
- •
Congenital muscular dystrophies
- •
Limb-girdle muscular dystrophy
- •
Infantile myotonic dystrophy
- •
Mitochondrial myopathy
- •
Brain stem anomaly
- •
Möbius syndrome
- •
Infantile botulism
- •
Seropositive and seronegative forms autoimmune myasthenia gravis a
a Not reported in the first year of life.
Older Patients
- •
Limb girdle or facioscapulohumeral dystrophy
- •
Seropositive and seronegative forms of autoimmune myasthenia gravis
- •
Mitochondrial myopathy
- •
Chronic fatigue syndrome
- •
Motor neuron disease
- •
Peripheral neuropathy b
b This diagnosis was made in some slow-channel CMS patients.
- •
Syringomyelia b
- •
Radial nerve palsy b
Genetic Diagnosis
A direct approach to diagnosis is Sanger sequencing based on available phenotypic clues (see Box 26.2 ). However, many CMS patients lack phenotypic clues. In that case mutation analysis can be based on frequencies of heretofore identified mutations in different proteins as shown in Table 26.1 . In large kinships, linkage analysis followed by Sanger sequencing of genes at the candidate chromosomal locus can be helpful. The use of microarray chips designed to detect variants in currently identified CMS disease genes will likely increase in the near future. Exome sequencing has now become a powerful tool for obtaining a genetic diagnosis when other approaches fail. However, this approach presently captures close to only ~85% of the entire exome and reads a smaller proportion of the exome at a high coverage. It also misses changes in noncoding DNA and large deletions or duplications, is less efficient at detecting dominant than recessive mutations, and is still very expensive. DNA from both parents, and whenever possible from other affected family members, should be analyzed. The bioinformatics analysis of the enormous amount of generated data remains challenging, and the putative pathogenic mutations must be confirmed by Sanger sequencing. The effects of novel nontruncating mutations can be assessed by available software programs, but the accuracy of these tools is not currently validated. Ideally, pathogenicity of novel variants should be evaluated by expression studies. Finally, pathogenic mutations may reside in novel genes whose function is yet unknown. Sequencing the whole genome is also feasible but the interpretation is even more expensive and complicated than that of exome sequencing.
Endplate AChR Deficiency Due to Low-Expressor Mutations in AChR Subunit Gene
- •
Ptosis, oculoparesis, weakness of limb muscles
Endplate acetylcholinesterase deficiency
- •
Repetitive CMAPs
- •
Refractoriness to cholinesterase inhibitors
- •
Delayed pupillary light reflexes in some cases
- •
Unresponsive to/worsened by cholinergic agonists; improved by ephedrine or albuterol
Slow-channel congenital myasthenic syndrome
- •
Repetitive CMAPs
- •
Selectively severe involvement of cervical and wrist and finger extensor muscles in most cases
- •
Dominant inheritance in most cases
- •
Worsened by cholinergic agonists; improved by long-lived AChR channel blockers
Endplate choline acetyltransferase deficiency
- •
Recurrent apneic episodes, spontaneous or with fever, vomiting, or excitement
- •
Variable myasthenic symptoms between acute episodes
- •
Stimulation at 10 Hz for 5 min causes marked decrease of the CMAP amplitude followed by slow recovery over 10 minutes. Rapid recovery in 2 to 3 minutes is not diagnostic
- •
EMG decrement at 2 Hz can be absent at rest but appears after stimulation at 10 Hz for 5 min, then disappears slowly over 10 minutes. Rapid recovery in 2 to 3 minutes is not specific
Rapsyn deficiency
- •
Multiple congenital joint contractures in one fourth of patients
- •
Increased weakness and respiratory insufficiency precipitated by intercurrent infections
- •
EMG decrement can be mild or absent
- •
Ophthalmoparesis in ~25%; strabismus relatively common
Dok-7 myasthenia
- •
Predominantly limb-girdle and axial distribution of weakness; mild facial weakness, and ptosis are common; normal ocular ductions in most patients
- •
Significant bulbar muscles involvement in some patients
- •
Worsened by cholinesterase inhibitors; responds to ephedrine or albuterol
- •
Can present with stridor and vocal cord paralysis in neonates and infants
GFPT1 myasthenia
- •
Predominantly limb-girdle and axial distribution of weakness.
- •
CK elevation in about one fourth
- •
Tubular aggregates in muscle in most patients; autophagic myopathy in some patients
- •
Improved by cholinergic agonists
DPAGT1 myasthenia
- •
Limb-girdle or diffuse weakness; ocular ductions spared
- •
Can be associated with congenital malformations and cognitive deficits
- •
Abnormal isolelectric focusing pattern of serum transferrin in some patients
- •
Tubular aggregates in muscle in most patients; autophagic myopathy in some patients
- •
Improved by cholinesterase agonists
Laminin β2 myasthenia
- •
Nephrotic syndrome, ocular abnormalities (Pierson syndrome)
- •
Refractoriness to cholinesterase inhibitors
Plectin deficiency myasthenia
- •
Epidermolysis bullosa simplex
- •
Refractoriness to cholinesterase inhibitors
Na-channel myasthenia
- •
Recurrent apneic episodes
- •
Suprathreshold EPPs fail to generate muscle fiber action potentials
Centronuclear myopathy-myasthenia
- •
High proportion of centronucleated muscle fibers as the predominant pathologic alteration
Index Cases at Mayo Clinic | % | |
---|---|---|
Presynaptic (5.6%) | ||
| 18 | 5.1 |
| 1 | 0.3 |
| 1 | 0.3 |
Synaptic basal lamina (13.3%) | ||
| 45 | 13 |
| 1 | 0.3 |
Postsynaptic (52%) | ||
| 62 | 18 |
| 118 | 33 |
| 1 | 0.3 |
| 2 | 0.6 |
Defects in endplate development and maintenance (29%) b | ||
| 1 | 0.3 |
| 1 | 0.3 |
| 51 | 14.5 |
| 35 | 10 |
| 11 | 3.1 |
| 2 | 0.6 |
| 1 | 0.3 |
Total | 351 | 100 |
b Mutations in ALG2 and ALG14 were identified at other medical centers.
In some CMS, and especially in novel syndromes, additional studies are required to elucidate the pathogenesis and to recommend appropriate therapy. Such studies include estimation of the number of AChRs per EP; histochemical, immunocytochemical, electron microscopy analysis of EP morphology; and electrophysiology assessment of EP function in vitro . The last includes microelectrode recording of EP potentials (EPPs) and currents, and single-channel patch-clamp recordings of currents flowing through single AChR channels.
Classification
The CMS can be classified according to inheritance, the clinical phenotype, the disease gene or protein, or the site of the defect (presynaptic, synaptic, or postsynaptic). Classification by inheritance is simple but not sufficiently informative: the slow-channel syndromes are caused by autosomal dominant gain-of-function mutations; all other CMS recognized to date are caused by autosomal recessive loss-of-function mutations. A classification according to the clinical phenotype, which includes the EMG findings, has merits because it can point to some syndromes (see Box 26.2 ), but it is still unsatisfactory because different presynaptic and postsynaptic syndromes cannot be reliably differentiated by clinical and EMG criteria. Classification by an altered EP-specific protein or gene is unsatisfactory because the disease gene of some CMS remains unidentified, and because different mutations of a given gene can cause different clinical phenotypes. A classification according to the site of the defect distinguishes among presynaptic, synaptic basal lamina, and postsynaptic syndromes and, by necessity, requires morphologic and sometimes in vitro electrophysiologic studies, but it is still not entirely satisfactory because disease genes that regulate EP development and maintenance are expressed at multiple anatomic sites.
The classification shown in Table 26.1 is based on the site of the defect and the effect on EP development and maintenance in 351 patients investigated at the Mayo Clinic. It shows that postsynaptic CMS are more frequent than presynaptic or synaptic CMS, and that most postsynaptic CMS are caused by deficiency or kinetic abnormality of the AChR. The distribution of the different types of CMS in any series could be biased by ease of identification of a given type of CMS by clinical criteria. Conversely, patients whose disease closely mimics a type of muscular dystrophy, congenital myopathy, or spinal muscular atrophy, may not be referred for further studies and may well remain undiagnosed. Also, identification and awareness of a given clinical phenotype (e.g. the slow-channel syndrome or EP AChE deficiency) promote identification of more patients of the same phenotype. The frequency of given types of CMS may also vary in different geographic areas and in different ethnic groups. In some closed communities the frequency of a given CMS is increased by a founder effect or by consanguineous marriages.
Presynaptic Congenital Myasthenic Syndrome
Endplate Choline Acetyltransferase Deficiency (Congenital Myasthenic Syndrome with Episodic Apnea)
Choline acetyltransferase (ChAT) catalyzes the resynthesis of ACh by transfer of an acetyl group from acetyl-CoA to choline in cholinergic neurons. Pathogenic mutations, alone or in combination, alter the expression, catalytic efficiency, or structural stability of the enzyme. When neuronal impulse flow is increased, as during exercise, the decreased rate of ACh synthesis progressively depletes the ACh content of the synaptic vesicles, and hence decreases the amplitude of the EPP and of the compound muscle action potential (CMAP). A useful clinical test for this disease consists of monitoring the EMG decrement and the CMAP amplitude during 10 Hz simulation over 5 minutes and for 10 to 15 minutes after stimulation. A nonspecific decrease below 50% of the potential from the baseline followed by return to the baseline in 2 to 3 minutes occurs in different CMS. In contrast, in patients with ChAT deficiency the recovery occurs slowly over 5 to 15 minutes. Therefore, the CMAP must be monitored for 10 to 15 minutes after 5 Hz stimulation.
Some patients present with hypotonia, bulbar paralysis, and apnea at birth ( Figure 26.2 ). Others are normal at birth and develop apneic attacks during infancy or childhood precipitated by infection, excitement, or no apparent cause. In some children an acute attack is followed by respiratory insufficiency that lasts for weeks. Few patients are apneic, respirator dependent, and paralyzed since birth, and some develop cerebral atrophy after episodes of hypoxemia. Others improve with age, but still have variable ptosis, ophthalmoparesis, fatigable weakness, and recurrent cyanotic episodes, and some complain only of mild to moderately severe fatigable weakness. The symptoms are worsened by exposure to cold because this further reduces the catalytic efficiency of the mutant enzyme.

Mutations with severe kinetic effects are located at or near the active-site tunnel or the substrate binding site of the enzyme, or exert their effect allosterically. Some mutations have no kinetic effects and express well but impair the thermal stability of ChAT by introducing a proline residue into an α-helix. Genotype–phenotype correlations are hindered in patients with biallelic mutations. Interestingly, however, in three very severely affected patients with lifelong apnea, permanent paralysis, and failure to respond to pyridostigmine, one of the two mutations was near the active-site of ChAT.
Treatment consists of prophylactic therapy with pyridostigmine. The parents should be provided with an inflatable rescue bag and a fitted mask, instructed in the intramuscular injection of neostigmine methylsulfate, and advised to install an apnea monitor in their home.
Paucity of Synaptic Vesicles Associated with Reduced Quantal Release
The clinical features closely mimic moderately severe to severe autoimmune myasthenia gravis, but the onset is at birth or in early infancy and tests for anti-AChR antibodies are negative. A specific diagnosis requires morphologic and electrophysiologic studies of the EP in a muscle biopsy specimen intact from origin to insertion. These studies reveal no EP AChR deficiency. A presynaptic defect is indicated by a severe decrease (to ~20% of normal) in the number of ACh quanta ( m ) released by nerve impulse. The decrease in m is due to a decrease in the number of readily releasable quanta ( n ), associated with a comparable decrease (to ~20% of normal) in the numerical density of the synaptic vesicles. The putative defect resides in the synthesis or axonal transport of vesicle precursors from the anterior horn cell to the nerve terminal, or is related to impaired recycling of the synaptic vesicles. Treatment consists of AChE inhibitors.
CMS Resembling the Lambert-Eaton Myasthenic Syndrome
In one young child reported with this syndrome, the CMAP amplitude was abnormally small but facilitated several-fold on tetanic stimulation, and the symptoms were improved by guanidine. The defect likely resides in a subunit of the presynaptic voltage-gated calcium channel or in a component of the synaptic vesicle release complex.
Synaptic Basal Lamina Associated CMS
EP Acetylcholinesterase Deficiency
The EP species of acetylcholinesterase (AChE) is an asymmetric enzyme composed of catalytic subunits encoded by ACHE T and a collagenic structural subunit encoded by COLQ that anchors the enzyme in the synaptic basal lamina. No spontaneous mutations have been observed in AChE T . The ColQ (collagen Q) protein is composed of three identical strands. The N-terminal residues of each strand bind a catalytic homotetramer. Mutations in this domain prevent ColQ association with the catalytic subunits and abolish enzyme activity. Frameshift or nonsense mutations in the collagenic midsection of ColQ produce a single-stranded insertion incompetent enzyme. Mutations of critical residues in the globular C-terminal region of ColQ prevent ColQ insertion into the synaptic basal lamina or hinder the triple-helical assembly of the ColQ strands ( Figure 26.3 ).

Patients typically present in the neonatal period or infancy with apnea and generalized weakness that persist through life ( Figure 26.4A ). In some patients the ocular ductions are spared and in some the pupillary light reflex is delayed. Milder cases associated with missense mutations in the C-terminal domain of ColQ may present later in childhood. The edrophonium test is negative and pyridostigmine causes increased secretions but has no effect on the weakness. Absence of AChE from the EP prolongs the lifetime of ACh in the synaptic cleft because each ACh binds multiple AChRs before leaving the synaptic space by diffusion. This prolongs the duration of the miniature EP potential (MEPP) and EPP, and when the EPP outlasts the absolute refractory period of the muscle fiber, it generates a second (or repetitive) muscle fiber action potential, reflected by a repetitive compound muscle action potential (CMAP) that is unaffected by edrophonium ( Figure 26.4B ). Cytochemical localization of AChE shows that the synaptic space is devoid of reaction product ( Figure 26.4C and D ).

Neuromuscular transmission is compromised by smallness of the nerve terminals and their encasement by Schwann cells, which reduces the number of releasable quanta, by an EP myopathy from cholinergic overactivity, and by desensitization and depolarization block of AChR at physiologic rates of stimulation. Smallness of nerve terminals and their encasement by Schwann cells are likely compensatory to protect the EP from overexposure to ACh.
Therapy is still unsatisfactory, but ephedrine and albuterol were noted to have a gradually developing beneficial effect.
CMS Associated with β2-Laminin Deficiency
β2-Laminin, encoded by LAMB2 , is a component of the basal lamina of different tissues and is highly expressed in kidney, eye, and the neuromuscular junction. Synaptic β2-laminin governs the appropriate alignment of the axon terminal with the postsynaptic region and, hence, pre- and postsynaptic trophic interactions. Defects in β2-laminin result in Pierson syndrome with renal and ocular malformations. A patient carrying heteroallelic missense and frameshift mutations in LAMB2 had Pierson syndrome associated with ocular, respiratory, and proximal limb muscle weakness. The renal defect was corrected by renal transplant at age 15 months. In vitro microelectrode studies revealed decreased quantal release by nerve impulse and a reduced MEPP amplitude. Electron microscopy showed abnormally small nerve terminals often encased by Schwann cells, accounting for the decreased quantal release. The synaptic space was widened and the junctional folds were simplified, accounting for the decreased MEPP amplitude.
Postsynaptic CMS
Most postsynaptic CMS stem from molecular defects in the muscle form of the nicotinic AChR. We therefore review the structure and kinetic properties of this species of AChR.
The Muscle Form of the Nicotinic Acetylcholine Receptor
The muscle form of nicotinic AChR is a pentameric transmembrane macromolecule with a subunit composition of α 2 βδε at adult EPs, and α 2 βδγ at fetal EPs and extrajunctional sites ( Figure 26.5 ). The genes encoding the α, δ, and γ subunits are at different loci on chromosome 2q; the genes encoding the β and ε subunits are at different loci on chromosome 17p. Neuronal AChRs are also pentameric, composed of moieties of two α (α 2 –α 9 ) and three β (β 2 –β 4 ) subunits, or exist as α homopentamers. Both muscle and neuronal AChRs are members of a superfamily of ligand-gated ion channels that also includes receptors for 5-hydroxytryptamine, γ-aminobutyric acid, and glycine. At the normal human EP AChR is concentrated on the crests of the junctional folds.

The five subunits of AChR are organized like barrel staves around a central cation-permeable channel ( Figure 26.5 ). Each subunit has an N-terminal extracellular domain that comprises approximately 50% of the primary sequence, four putative transmembrane domains (M1–M4), and a small C-terminal extracellular domain. M2, which lines the ion channel, forms an α-helix interrupted by a short stretch of β-sheet. C-terminal residues of the M1 domain may also contribute to the channel lumen in the resting state. The transmembrane domains are connected by an extracellular M2/M3 linker and by intracellular M1/M2 and M3/M4 linkers. The M3/M4 linker forms a long cytoplasmic loop that likely serves as an attachment site for cytoskeletal elements, contains phosphorylation sites that may be important for desensitization, and harbors residues that keep the gating properties of the ion channel consistent. Each AChR has two ACh-binding pockets positioned at the α/ε (or α/γ) and α/δ interfaces. Residues contributing to the binding pocket appear on three peptide loops on the α subunit and four peptide loops of the δ, ε, and γ subunits. Crystallography studies indicate that each ACh-binding pocket is open to the extracellular space.
Two ACh molecules (A) bind to the receptor (R) with association rates k +1 and k +2 and dissociate from it with rates k −2 and k −1 . The double-liganded receptor (A2R) opens with rate β, and the open receptor (A2R*) closes with rate α. A channel opening episode, or opening burst, consists of one or more openings interrupted by brief closures due to oscillations of the diliganded receptor between the open and closed state. The above scheme predicts that the mean duration of channel-opening episode bursts is approximately (1+β/k −2 )/α. This value also corresponds to the decay time constant of the miniature EP current (MEPC) evoked by a single quantum of ACh.
Slow-Channel Syndrome
This syndrome arises from dominant gain of function mutations that occur in extracellular or transmembrane domains of each AChR subunit. The mutations enhance the agonist affinity or the gating efficiency of the receptor. Either mechanism prolongs the single-channel currents, EP currents, and EPPs. As in EP AChE deficiency, when the length of the EPP exceeds the absolute refractory period of the muscle fiber, it triggers a repetitive CMAP ( Figure 26.6A–C ), but unlike in AChE deficiency, the repetitive response is accentuated by edrophonium. Also, at physiologic rates of stimulation, each prolonged EPP arises in the wake of the preceding EPP causing a progressive depolarization block of the postsynaptic membrane. The prolonged EP currents and leakiness of the ion channels even in the closed state result in Ca 2+ overloading of the postsynaptic region ( Figure 26.6 D and E ) and an EP myopathy ( Figure 26.6F ). The safety margin of neuromuscular transmission is compromised by a depolarization block, by desensitization of the AChR during physiologic activity, and by the endplate myopathy that causes loss of AChR from the junctional folds and alters the EP geometry.
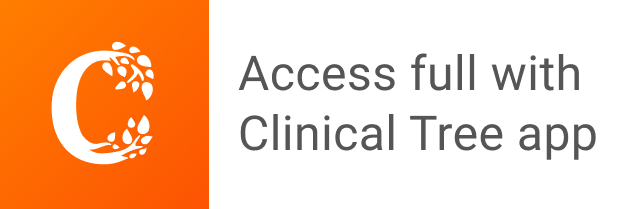