Keywords
Mitochondrial DNA, nuclear DNA, maternal inheritance, heteroplasmy, intergenomic communication, membrane phospholipids, mitochondrial dynamics, mtDNA depletion, single mtDNA deletions, multiple mtDNA deletions, oxidative stress
Introduction
Historical Background
The history of mitochondria can be traced back at least to 1850 when Kolliker described a regular array of subcellular particles in muscle sarcomeres. Fifty-two years were to pass before Luft and colleagues implicated mitochondria as the cause of a human disease. A 39-year-old woman had florid symptoms of hypermetabolism thought initially to be due to thyroid disease. However, antithyroid medication and partial thyroidectomy were ineffective. Studies of biopsied muscle would prove to be more informative. Isolated mitochondria were loosely coupled in vitro by polarographic measurements, and the number of organelles was vastly increased by morphological criteria. Many mitochondria were abnormally large with tightly packed cristae ( Figure 41.1 ). Later, Shy and colleagues would use the terms pleoconial and megaconial when describing similar mitochondrial abnormalities in the biopsied skeletal muscle specimens from some children with limb weakness.

The term mitochondrion was coined by Benda in 1898. The specialized function and the unique structure of mitochondria emerged in the 20th century. Keilin described the cytochrome system and Warburg found evidence of a terminal oxidase system. He also observed that nicotine adenine dinucleotide behaved as a hydrogen acceptor in oxidation-reduction reactions. Flavoproteins were found to act as intermediary carriers of electrons between the reduced form of nicotine adenine dinucleotide and cytochrome systems. Krebs and Kornberg discovered that phosphorylation in aerobic systems depended on the consumption of oxygen, hence the concept of “oxidative phosphorylation.”
Krebs and Kornberg continued their seminal observations in the 1930s, showing that citrate was formed from pyruvate and oxaloacetate. These observations led to the discovery of the tricarboxylic acid cycle (the TCA cycle, now better known as Krebs cycle), which converted pyruvate to carbon dioxide and water. These investigators and others showed that oxidation of intermediates of the tricarboxylic acid cycle was coupled to the generation of adenosine triphosphate.
The importance of the mitochondria as the source of cellular energy was being established by these seminal observations. Additional experiments explained the relationships between fuel combustion to carbon dioxide and water, the generation of reducing equivalents in the Krebs cycle, and the re-oxidation of these equivalents, NADH and FADH 2 , in the respiratory chain coupled with the synthesis of ATP. The concept of oxidation-phosphorylation was established and the chemiosmotic hypothesis of Mitchell in 1961 proposed the proton-motive force as the primary source of energy for ATP synthesis in complex V. The rudimentary structure of the respiratory chain now was complete with the five enzyme complexes. More critical observations would follow over the next 50 years, characterizing in greater detail the subunit structure of the respiratory chain complexes, the critical shuttling roles of coenzyme Q10 and cytochrome c, and the dual roles of nuclear DNA and mitochondrial DNA in the synthesis of the many subunits that composed the five enzyme complexes.
In parallel with these brilliant biochemical observations, a series of studies was being published describing the ultrastructural characteristics of the mitochondrion. Four components of the organelle were characterized: the outer and inner membranes, the intermembranous space, and the inner mitochondrial compartment matrix. A series of studies subsequently demonstrated that the inner mitochondrial membrane was largely impermeable to molecules of all sizes, and special adaptations were necessary for the translocation of metabolites and proteins from the intermembranous space to the mitochondrial matrix. These observations set the stage for an understanding of the complex importation processes that are necessary both to translocate solutes across the inner mitochondrial membrane and to move proteins from the cytoplasmic environment to the intramitochondrial environment. Protein translocation is elaborate and energy-dependent, requiring the proteins to be unfolded before traversing the mitochondrial outer and inner membranes and then refolded after entering the mitochondrial matrix. The leader sequence that guides the protein through the mitochondrial membrane to the matrix is cleaved by a mitochondrial-specific protease. Only 13 proteins are synthesized within the mitochondrial matrix by protein-coding genes located in the mitochondrial genome.
In 1963, two important observations were made simultaneously and independently. The first observation described the presence of intramitochondrial fibers with DNA characteristics. Twenty-five years would elapse before this observation would take on clinical significance. The second observation was the description of abnormal mitochondria under the light microscope: Engel and Cunningham exploited a modification of the Gomori trichrome stain and found conglomerations of abnormal organelles in the cross section of muscle fibers that they called “ragged-red fibers” (RRF) ( Figure 41.1 ). The RRF almost invariably contained structurally abnormal mitochondria as seen under the electron microscope. These morphological criteria later were displaced by biochemical and genetic criteria for the classification of mitochondrial diseases.
Early morphological observations gradually gave rise to another fundamental biological principle related to mitochondria. Mitochondrial ribosomes resemble bacterial ribosomes under the electron microscope. This observation led Ephrussi and colleagues in 1949 to suggest that mitochondrial morphogenesis was controlled by extrachromosomal or cytoplasmic genetic factors. The observation of a yeast mutation causing a deficiency of respiratory function and inherited in a non-Mendelian fashion was central to the development of the unique theory put forward by Ephrussi and colleagues. Schatz et al. in 1964 found DNA in yeast mitochondria and this observation led him to propose that the yeast organelle might be self-replicating and relatively independent of the nuclear genome. A series of observations followed and culminated in the report by Fine in 1978 that all mitochondrial DNA was derived from the unfertilized ovum. This hypothesis was validated two years later by Giles and colleagues when they were able to trace the inheritance of a mitochondrial DNA polymorphism in a 33-member three-generation family. The typical mitochondrial DNA cleavage pattern and the atypical mitochondrial DNA cleavage pattern could be traced from one generation to the next, clearly documenting the non-Mendelian pattern of maternal inheritance. Later, it would be shown that clinical conditions related to mitochondrial DNA (mtDNA) mutations were transmitted from the mother to all of her male and female progeny, but only the daughters would be able to pass the condition to succeeding generations. This genetic profile was similar to Mendelian inheritance including autosomal dominant and X-linked patterns, but differed from Mendelian inheritance because both genders were equally affected and there was no evidence of father-to-child transmission. Wallace and colleagues later would add two other important concepts to explain the variable expression of maternally inherited genetic defects: namely, replicative segregation and threshold effect.
The historical stage now was set for the two dramatic publications that appeared in 1988 and that introduced the medical community to the presence of mitochondrial DNA mutations as a new cause of human diseases. Holt et al. reported large-scale deletions of mitochondrial DNA in patients with a mitochondrial myopathy and Wallace et al. described a point mutation in the mitochondrial protein-coding gene for subunit 4 of complex I ( ND4 ) in a family with Leber’s hereditary optic neuropathy (LHON). More than 200 additional mitochondrial DNA point mutations would be described over the next 25 years in addition to numerous mitochondrial DNA large-scale rearrangements. The number of mitochondrial DNA mutations continues to increase, and now exceeds 250 at the time of this chapter revision.
These mutations affect all aspects of the mitochondrial genome, which had been fully characterized and sequenced in 1981 by Anderson and colleagues. The double-stranded molecule contains 16,569 base pairs. Thirty-seven genes are represented including two ribosomal RNAs, 22 transfer RNAs, and 13 protein-coding genes. This molecular machinery allows for the intramitochondrial synthesis of 13 subunits of the respiratory chain. Seven of these subunits are located in complex I, one in complex III, three in complex IV, and two in complex V ( Figure 41.2 ). The simplicity of the mitochondrial genome is shown in Figure 41.2 and is characterized by the circularity, the absence of introns, and the presence of one asymmetric replication origin.

Epidemiology
Epidemiological studies have confirmed the assumption of clinicians that this group of diseases is underestimated. In fact, epidemiological studies limited to mtDNA-related disorders in adults have shown a minimum prevalence of approximately 1 in 5000, making them among the most common genetic disorders and a major burden for society. More interesting to pediatricians, screening of umbilical cord blood for the 10 most common pathogenic mtDNA point mutations revealed that around 1 in 200 infants harbors a mutation. As the prevalence of mtDNA-related disorders is far less than 1 in 200, it must be concluded that the mutation load in most carriers remains below the pathogenic threshold (see below). This concept is also in keeping with the results of a supersensitive next-generation sequencing study showing that low-level changes (0.2–2.0% heteroplasmy [the coexistence of mutated and wild-type mtDNAs within a cell—see the following]) are present in blood and skeletal muscle of clinically unaffected individuals, a phenomenon dubbed “universal heteroplasmy.” The possibility exists, therefore, that clinically silent mtDNA mutations could expand over time and manifest as de novo mitochondrial diseases.
Beginning in 1995, mutations in nuclear genes affecting the respiratory chain were identified at an escalating pace, largely thanks to linkage analysis, homozygosity mapping, monochromosomal transfer techniques, gene complementation studies, and, more recently, whole exome or mito-exome sequencing. As Mendelian mitochondrial disorders are more common in infants and children than in adults, as exemplified by Leigh syndrome (LS), next-generation sequencing is particularly important for the molecular diagnosis of pediatric disorders.
Classification
Our earlier classification of mitochondrial diseases was based on the biochemical site of dysfunction, for example substrate transport, substrate utilization, Krebs cycle, respiratory chain, and protein importation. However, this scheme proved inadequate and clumsy when assessing patients with various clinical manifestations. The discovery in 1988 of disease-causing mtDNA mutations and, starting in 1995, the description of myriad diverse Mendelian mitochondrial disorders, forced a revision of the classification, which is today rationally and comprehensively based on molecular criteria.
Keeping in mind that a generally accepted definition of mitochondrial diseases restricts these diseases to defects of the respiratory chain and oxidative phosphorylation (OXPHOS), they fall broadly into two categories: those due to mutations in mtDNA, which cause maternally inherited or sporadic disorders; and those due to mutations in nDNA, which show Mendelian inheritance.
A more practical way of classifying these disorders is to divide them into three groups: mtDNA defects; defects of nDNA that affect the respiratory chain directly or indirectly; and defects of mtDNA maintenance (also known as defects of intergenomic communication) ( Table 41.1 ). We separate out the defects of mtDNA maintenance for two reasons: (i) they involve the mitochondrial genome directly, causing multiple mtDNA deletions or mtDNA depletion; and (ii) although unequivocally Mendelian with regard to inheritance, these disorders share much of the clinical heterogeneity of primary mtDNA-related diseases because the polyploid mtDNA is involved in both conditions.
Mitochondrial DNA (mtDNA)-Related Diseases (Maternal or Sporadic) | Nuclear DNA (nDNA)-Related Diseases (Mendelian) |
---|---|
|
|
|
|
| |
| |
| |
|
Pathogenesis and Genetics
There are two main disturbances of cell function associated with mitochondrial diseases: failure to generate sufficient ATP to carry out cellular work; and failure to neutralize reactive oxygen species (ROS) with resulting damage to structural elements of the cell. All categories of mitochondrial diseases, as shown in Table 41.1 , impair ATP production. Clinically, these patients exhibit classical symptoms of energy failure such as weakness, hypotonia, reduced physical stamina, muscle atrophy, heart failure, and limitation of eye movements with ptosis. Developmental processes also may suffer as shown by failure to thrive with short stature, reduced muscle bulk, and deceleration of head growth.
Accumulation of ROS damages tissue membranes, promotes genetic mutations, and contributes to cell death. Symptoms associated with this insidious tissue damage are more difficult to appreciate in a correlative sense but reflect the overall degenerative process. The failing heart, muscular atrophy, and basal ganglia damage likely reflect this microchemical attack on tissue structures, suggesting that the pathogenesis of human mitochondrial diseases directly affecting oxidation-phosphorylation mechanisms is intensified by the combination of energy failure and the accumulation of ROS.
Before proceeding with the description of mitochondrial diseases, it may be useful to remind the readers of the bona fide mitochondrial diseases that do not belong to this category because they are not due to defects of the respiratory chain and OXPHOS. They belong in three major categories: defects of fatty acid oxidation (FAO); defects of pyruvate metabolism; and defects of the Krebs cycle (except for succinate dehydrogenase [SDH, complex II], which belongs both to the Krebs cycle and to the respiratory chain) ( Figure 41.3 ). FAO defects are discussed in Chapter 40 .

The molecular genetics of mitochondrial diseases is complex because it is determined by two genomes, nDNA and mtDNA. The respiratory chain is composed of five complexes containing about 85 subunits, all of which are encoded by nDNA except 13 that are encoded by mtDNA ( Figure 41.2 ). As a result, a defect of the respiratory chain may be genetically determined as an autosomal trait or as a maternal trait. The autosomal conditions may be dominant, recessive, or X-linked traits. Maternally transmitted traits are non-Mendelian and obey the rules of mitochondrial genetics. There are three biological rules that explain mitochondrial genetics. The first is maternal inheritance . Sperm mtDNA is actively degraded after fertilization; as a result, all mtDNA is maternally inherited. Mitochondrial DNA mutations, therefore, are transmitted from mother to her male and female progeny, but only her daughters will transmit the mutation to their children.
The second rule is heteroplasmy , with the related concept of threshold effect . Each mitochondrion contains two to ten mitochondrial DNA copies, and each cell contains hundreds or thousands of mitochondria. Therefore, any given cell may contain as many as several thousand mitochondrial DNA copies. If an mtDNA mutation exists, some of the molecules will harbor the mutation whereas other molecules will be normal. This mixture of mutated and wild-type mtDNAs is referred to as heteroplasmy. Despite recent evidence of “universal heteroplasmy” (see above), for all practical purposes all mtDNAs in normal cells are wild-type, a condition called homoplasmy. An increasing percentage of mutated mtDNAs threatens the biological integrity of the cell until the mutation load reaches a theoretical threshold and the cellular phenotype is transformed from normal to diseased. Pathogenic mtDNA mutations usually are heteroplasmic whereas neutral polymorphisms (nondeleterious mtDNA mutations) are homoplasmic, but there are many exceptions to this rule, notably the three mutations commonly associated with LHON. As the threshold varies inversely with the oxidative demands of the tissue, oxidative-rich tissues are more vulnerable to the presence of pathogenic mtDNA mutations.
The third rule is mitotic segregation , which stipulates that the proportion of mutant mtDNAs can shift in daughter cells at division. This stochastic shift in mutated mtDNAs during mitosis can result in segregation of the mutation and is invoked as an explanation for the phenotypic heterogeneity among patients with the same mtDNA point mutation, including siblings. For example, the m.3243A>G mutation, commonly referred to as the MELAS mutation, may produce several different phenotypes, one reflecting the classical MELAS presentation (mitochondrial encephalomyopathy, lactic acidosis, and stroke-like episodes), others reflecting different clinical pictures such as progressive external ophthalmoplegia (PEO), myopathy, cardiomyopathy, and diabetes mellitus and deafness (see Case Example 41.1 ). Presumably, these phenotypic differences are determined by the segregation of the A3243G point mutation during mitosis, although the influence of modifying mtDNA or nDNA genes cannot be excluded. Other examples of phenotypic heterogeneity and genotypic homogeneity are shown in the morbidity map of the human mitochondrial genome ( Figure 41.4 ). This figure also demonstrates examples of phenotypic homogeneity and genotypic heterogeneity, including the diverse point mutations resulting in Leigh syndrome (LS) or in MELAS.
A 5-year-old girl lost consciousness for 30 minutes after strenuous exercise. She also had multiple episodes of dimmed vision lasting a few minutes. Pregnancy and delivery had been normal and she walked at 1 year. Weakness of the legs had become apparent at 2 years when she had difficulty climbing stairs. At 6 years, episodes of right-sided paresthesias after exertion lasted 30–60 minutes and were followed by blindness. One episode was followed by a generalized tonic-clonic seizure and phenobarbital therapy was started. Postictally, there was hemiparesis that improved after 3 days. A second right focal seizure was followed by a hemiparesis for 5 days. A third generalized seizure was accompanied by blindness, which was thought to be cortical in origin because pupillary reactions to light and fundoscopic examination were normal. Audiogram was normal at age 5 years but showed neurosensory hearing loss at age 9 years. The disorder progressed and at 9 years she was severely demented and deaf, speaking only in short phrases. At 10 years, she died in congestive heart failure. Family history showed that a 17-year-old brother had short stature, mental retardation, and hearing loss. Her older sister was normal and so were her mother and maternal grandmother. On repeated occasions, venous blood obtained without a tourniquet was at least 4 times higher than normal. Brain CT scan at age 7 years showed mild cerebellar atrophy. A muscle biopsy at age 7 years showed numerous COX-positive RRF. Biochemical studies in muscle homogenate showed markedly decreased activities of all respiratory chain complexes except complex II (succinate dehydrogenase), which was actually higher than normal. Citrate synthase activity was increased two-fold. Molecular genetic analysis showed the m.3243A>G mutation in the Leu(UUR) of mtDNA. Mutation loads in blood ranged from 55% in the proposita to 14% in the brother, 59% in the mother, and 15% in the sister. The mutation load in the patient’s muscle was 83%. At autopsy, there was mild atrophy of the cerebral hemispheres with countless small cortical infarcts, especially in the occipital lobes and in the posterior portions of the temporal lobes. The cortical lesions were often laminar and involved the three deepest layers.
Comment
This patient was the first reported case of MELAS harboring the “common” MELAS-3243 mutation in mtDNA, and it was revisited in 1992 by Hirano et al. The presentation was typical, with normal initial development followed by recurrent and partially reversible stroke-like episodes, often triggered by seizures. Involvement of the occipital lobe with cortical blindness is common. Maternal inheritance was not obvious phenotypically in this family, but it became evident at the molecular level. The mutational load in blood did not accurately reflect clinical severity, as is often the case. Among easily available tissues, urinary sediment is a better molecular marker of severity. The fact that RRF were COX-positive is a peculiar feature of MELAS among mtDNA-related disorders. The pattern of muscle biochemistry is typical of a mtDNA mutation affecting mitochondrial protein synthesis: increased activities of SDH and citrate synthase (both encoded by nDNA) and variously decreased activities of complexes containing mtDNA-encoded subunits.

Clinical Features
There are over 250 pathogenic mtDNA point mutations, most of which affect mitochondrial protein synthesis while a smaller number affect protein-coding genes. Pure myopathies and neuropathies are unusual, but neuromuscular symptoms are common as part of multisystemic phenotypes. Multisystemic disorders are illustrated by Kearns-Sayre syndrome (KSS), Pearson syndrome, MELAS, myoclonus epilepsy and RRF (MERRF), and neuropathy, ataxia, and retinitis pigmentosa/maternally inherited LS (NARP/MILS) ( Table 41.2 ). Two of these diseases (KSS and Pearson syndrome) are due to large-scale deletions; two (MELAS and MERRF) are caused by tRNA mutations that impair mitochondrial protein synthesis globally; and the remaining two diseases (NARP and MILS) are due to mutations in a single protein-coding gene.
Tissue | Symptom/Sign | Δ-mtDNA | tRNA | ATPase6 | |||
---|---|---|---|---|---|---|---|
KSS | Pearson | MERRF | MELAS | NARP | MILS | ||
Central nervous system | Seizures | − | − | + | + | − | + |
Ataxia | + | − | + | + | + | +/− | |
Myoclonus | − | − | + | +/− | − | − | |
Psychomotor retardation | − | − | − | − | − | + | |
Psychomotor regression | + | − | +/− | + | − | − | |
Hemiparesis/hemianopia | − | − | − | + | − | − | |
Cortical blindness | − | − | − | + | − | − | |
Migraine-like headaches | − | − | − | + | − | − | |
Dystonia | − | − | − | + | − | + | |
Peripheral nervous system | Peripheral neuropathy | +/− | − | +/− | +/− | + | − |
Muscle | Weakness | + | − | + | + | + | + |
Ophthalmoplegia | + | +/− | − | − | − | − | |
Ptosis | + | − | − | − | − | − | |
Eye | Pigmentory retinopathy | + | − | − | − | + | +/− |
Optic atrophy | − | − | − | − | +/− | +/− | |
Cataracts | − | − | − | − | − | − | |
Blood | Sideroblastic anemia | +/− | + | − | − | − | − |
Endocrine | Diabetes mellitus | +/− | − | − | +/− | − | − |
Short stature | + | − | + | + | − | − | |
Hypoparathyroidism | +/− | − | − | − | − | − | |
Heart | Conduction block | +/− | − | − | +/− | − | − |
Cardiomyopathy | +/− | − | − | +/− | − | +/− | |
Gastrointestinal | Exocrine pancreas dysfunction | +/− | + | − | − | − | − |
Intestinal pseudo-obstruction | − | − | − | − | − | − |
Mitochondrial genetics provides some explanation for the clinical heterogeneity of these diseases. For example, the degree of heteroplasmy of the same mutation in the gene encoding ATPase 6 (m.8993T>G) is clearly related to the severity and age at onset of NARP, a disorder of young adults with ~70% mutation load, and MILS, a severe neurodegenerative disease of infancy or young children with mutation loads >90%.
A conundrum that lies at the heart of mitochondrial genetics regards the pathogenesis of typical heteroplasmic diseases. Simply put, why is KSS different from MELAS and MELAS different from MERRF, when all three conditions result from impaired mitochondrial protein synthesis and ATP production? It would be logical to expect phenotypic homogeneity rather than syndromic individuality and yet—despite occasional overlapping presentations—this is not the case. There is immunohistochemical evidence that the different mutations that characterize the three syndromes have distinct spatial distribution in the brain: the single deletions of KSS are more abundant in the choroid plexus; the most common m.8344A>G MERRF mutation predominates in dentate and olivary nuclei; and the typical m.3443A>G mutation abounds in subpial arterioles. Although these preferential brain localizations do correlate with typical symptomatology (e.g. MELAS is ultimately a mitochondrial angiopathy), the question then becomes what directs the different mutant mtDNAs to selective regions of the brain.
Diseases Due to mtDNA Mutations
Large-Scale Rearrangements of mtDNA
These genetic abnormalities include single large-scale deletions and duplications of mtDNA, i.e. each patient harbors a single species of partially deleted mtDNA. The rearrangements generally are several thousand base pairs in length ( Figure 41.5 ).

Major Deletions and Duplications
Large-scale partial deletions and duplications of mtDNA generally occur spontaneously. More than 200 species of rearranged mtDNAs have been described since the original report by Holt et al. in 1988. Shortly thereafter, investigators established the association of single major deletions with KSS and sporadic PEO. The most frequently encountered deletion, termed the “common deletion,” spans 4977 base pairs. The common deletion, like most other major deletions, removes both structural and synthetic genes, thus impairing mitochondrial protein synthesis. The following disorders are associated with single large-scale rearrangements of mtDNA.
Kearns-Sayre Syndrome
KSS is defined by onset before age 20 years, progressive ophthalmoplegia, and pigmentary retinopathy. In addition to this triad of symptoms, at least one additional symptom must be present: cardiac conduction block, cerebellar syndrome, or cerebrospinal fluid (CSF) protein concentration >100 mg/dL. Patients with KSS exhibit many of the classical signs and symptoms of mitochondrial DNA-related disorders such as short stature, dementia, sensorineural hearing loss, isolated endocrinopathies including diabetes mellitus, hypothyroidism, hypoparathyroidism, and growth hormone deficiency. Surprisingly, seizures are very infrequent and commonly are associated with hypoparathyroidism. Brain MRI shows diffuse white matter abnormalities ( Figure 41.6D , KSS). Muscle histology is abnormal, with ragged-red fibers and a mosaic pattern of cytochrome c oxidase (COX)-negative fibers. Mitochondrial DNA deletions are easily demonstrated in muscle, and are less likely to be found in blood DNA extracts.

KSS almost always is sporadic and a multicenter retrospective study of 226 families has shown that the risk of a carrier woman to have affected children is very small but finite (1 in 24 births) while disproving the idea that the risk increases with maternal age. There are rare examples of women with the symptoms of KSS who have had children with Pearson’s marrow/pancreas syndrome and the same mitochondrial DNA deletion as the mother.
Pearson’s Syndrome
Pearson’s syndrome is a medical disorder affecting infants, and causing a severe sideroblastic anemia, pancytopenia, and exocrine pancreas dysfunction. The hematological disorder often is life-threatening, but those patients who survive later develop the symptoms of KSS.
Sporadic PEO with RRF
These patients manifest PEO, ptosis, and pharyngeal and proximal limb weakness, usually beginning in adolescence or young adulthood.
Duplications of mtDNA have been reported in patients with KSS. Maternally inherited duplications have been associated with a syndrome including cerebellar ataxia, proximal renal tubular nephrosis, and diabetes mellitus. Brockington et al. have suggested that duplications may be the underlying cause of all maternally transmitted deletions of mitochondrial DNA including the approximately 10 kilobase deletion observed in a family with maternally transmitted diabetes mellitus and deafness.
In summary, sporadic single mtDNA deletions and/or duplications may be associated with the classical phenotype of Kearns-Sayre syndrome and with other phenotypes including progressive external ophthalmoplegia, Pearson’s syndrome, and renal tubular nephrosis with diabetes mellitus and ataxia. Limb weakness is evident but may be a less conspicuous clinical finding in these phenotypes.
Point Mutations in mtDNA
Over 250 pathogenic point mutations pepper the small mtDNA molecule ( Figure 41.4 ). We will consider separately those that impair mitochondrial protein synthesis globally (mutations in rRNA and in tRNA genes) and those that affect protein-coding genes.
Point Mutations in rRNA and tRNA Genes
Deleterious mutations in rRNA genes are extremely rare, accounting for only 2% of all mtDNA pathogenic mutations (the coding capacity of the two rRNA genes is 15%). The phenotypes include aminoglycoside-induced deafness and dilated cardiomyopathy.
The phenotypes associated with point mutations in tRNA genes commonly are multisystemic and include classic clinical syndromes, such as MELAS, MERRF, NARP/MILS ( Table 41.2 ), LHON, and several other disorders. Cardiomyopathy and myopathy frequently keep company with encephalopathy in these multisystemic phenotypes. Less frequently, myopathy may dominate the clinical picture. These “pure myopathies” can be explained by at least two mechanisms: (i) somatic mutations that occur de novo in the mitochondrial genome of the oocyte or the embryo but affect only myoblasts after germ-layer differentiation, thus sparing other tissues; or (ii) skewed heteroplasmy, a situation in which the tRNA mutation is ubiquitous but the mutation load surpasses the pathogenic threshold only in muscle.
MELAS was first described in 1984 as a clinical syndrome manifested by mitochondrial encephalomyopathy with lactic acidosis and stroke-like episodes and probably it is the second most common maternally inherited mitochondrial disease after LHON. Clinically, it is defined by the stroke-like episodes that cause focal brain injury. Seizures are present in nearly 100% of patients and a subacute dementia develops rapidly after the initial clinical presentation. The blood and CSF lactic acid concentrations are elevated and RRF are present in the biopsied muscle tissue. Patients frequently have migraine-like headaches, recurrent vomiting, exercise intolerance, limb weakness and short stature. The stroke-like episodes commonly cause hemiparesis, hemianopia, or cortical blindness, and focal seizures often herald the onset of the stroke-like episodes. The etiology of the stroke-like episodes is uncertain but the prevailing evidence suggests that these episodes represent focal brain injury rather than vaso-occlusive disease. Microscopic studies have documented a mitochondrial angiopathy with increased numbers of enlarged mitochondria that are strongly positive for succinate dehydrogenase in the endothelial cells of both muscle and intracerebral small vessels. Diffusion weighted MRI studies have shown evidence for vasogenic rather than cytotoxic edema, consistent with a focal breakdown of the blood-brain barrier ( Figure 41.6C , MELAS). This observation suggests a therapeutic role for corticosteroids in the treatment of the acute stroke-like episode, expecting that the corticosteroids would mitigate the vasogenic edema in the same way that corticosteroids benefit the edema associated with brain tumors. There have been no clinical trials to date to examine this speculation.
Phenotypic heterogeneity has been well documented in patients harboring the A3243G point mutation in the tRNA Leu(UUR) gene. These clinical variations may include gastrointestinal features with malabsorption or dysmotility, cardiomyopathy, extrapyramidal syndromes, cluster headaches, pancreatitis, LS, a relatively pure myopathy with PEO, and a number of overlap syndromes. The cardiomyopathy may progress from a hypertrophic to a dilated type, and accessory conduction pathway syndromes may be associated, such as the Wolff-Parkinson-White syndrome.
Dermatological evaluation also has revealed an increased incidence of vitiligo, seborrheic eczema and atopy, and fragility of blood vessels with resulting localized bleeding and ecchymoses. Psychiatric symptomatology is prominent and underreported in patients with the MELAS mutation.
Eighty percent of patients fulfilling the clinical criteria for MELAS harbor the A3243G point mutation. About a dozen other genotypes are associated with the MELAS phenotype (see Table 41.2 ). Ragged-red fibers are present in the biopsied muscle tissue regardless of whether the mutation involves a synthetic gene or a protein-coding gene. Most of the RRF are COX-positive. Another unusual histological feature is the presence of strongly SDH-reactive blood vessels, the so-called SSRBV.
Maternally Inherited PEO
In this genetically determined condition, PEO is the predominant clinical feature. Other less consistent signs include hearing loss, endocrinopathy, heart block, cerebellar ataxia, and pigmentary retinopathy. Lactic acidosis is present but not as frequent or as striking as in patients with MELAS. Muscle biopsy shows RRF with more COX-negative fibers than in typical MELAS. Individual muscle fibers from patients with PEO have shown a higher abundance of mutated mtDNA than the COX-positive RRF in patients with MELAS.
The maternal inheritance pattern distinguishes this PEO phenotype from sporadic PEO associated with single large-scale mtDNA deletions (see above). The most common cause of maternally inherited PEO is the A3243G point mutation that is typically associated with the MELAS phenotype. Other mtDNA point mutations associated with this phenotype involve tRNA ILe , tRNA Asn , tRNA Tyr (see Table 41.2 ).
Isolated Myopathy without PEO
Although infrequent, pure myopathies have been associated with tRNA mutations: they often affect preferentially respiratory muscles and some have been described in children. They can be inherited maternally or be sporadic traits due to de novo mutations and the isolated involvement of skeletal muscle is probably due to extremely skewed heteroplasmic distribution of the pathogenic mutation (see above).
An unusual presentation is a severe but spontaneously reversible infantile mitochondrial myopathy characterized biochemically and histochemically by profound deficiency of COX in muscle. A homoplasmic mutation (m.14674T>C in tRNA Glu ), initially considered a neutral polymorphism, was found in 17 patients from 12 families of various ethnic origins, and the same mutation (or a T>G mutation at the same site) was confirmed in eight Japanese patients. Although the pathogenicity of the mutation was established through high-resolution northern blot analyses of muscle biopsies during the symptomatic phase and through biochemical studies of cybrid cell lines, the mutation per se did not explain the tissue specificity or the reversibility of the clinical and biochemical phenotype. It took 4 more years to discover that tRNA Glu has to be 2-thiouridylated by TRMU (5-methylaminomethyl-2-thiouridylate methyltransferase) and that TRMU is downregulated during the symptomatic phase of the disease, thus exacerbating the effects of the tRNA Glu mutation. This is in keeping with previous observations that reversible forms of infantile myopathy and hepatopathy were associated with mutations in TRMU and changes the classification of the reversible COX-deficiency myopathy from a primary mtDNA defect to a defect of mtDNA translation (see below).
Myoclonus Epilepsy with Ragged-red Fibers
Myoclonus epilepsy with ragged-red fibers (MERFF) is manifested by myoclonus, ataxia and seizures. but it may have protean manifestations. Myoclonus often is the presenting symptom and may be precipitated by action, noise, or photic stimulation. Muscle weakness and exercise intolerance are common. RRF are seen in muscle biopsy and the affected fibers are COX negative.
Additional clinical features have been described in patients and intrafamilial phenotypic variability is recognized. Added clinical features include hearing loss, cognitive impairment, multiple lipomas, neuropathy, and ptosis/ophthalmoplegia. A subtle, slowly progressive dementia may occur in patients, often after several decades, but cognition is relatively spared in contrast to the MELAS phenotype. Phenotypic variability within single families has been described with clinical features ranging from the classic MERRF phenotype to spinocerebellar degeneration, typical Charcot-Marie-Tooth disease, and LS.
MERRF was the first well-defined human disease in which maternal inheritance was clearly demonstrated, thus suggesting a defect in mtDNA and the first disorder in which a molecular disorder was associated with epilepsy. Approximately 80% of patients with MERRF have the m.8344A>G point mutation involving the tRNA Lys gene. Other mutations in this gene have also been associated with the MERRF phenotype, including the T8356C and the G8363C point mutations. Peculiar presentations of the m.8344A>G mutation include a sporadic case of histiocytoid cardiomyopathy with death at 11 months and a case with myopathy and multiple lipomas but also strokelike episodes and severe gastrointestinal dysfunction, more akin to MELAS than to MERRF. An overlap syndrome of MERRF and KSS was due to a mutation in the tRNA Leu(UUR) gene.
Point Mutations in Protein-Coding Genes
NARP/MILS
These syndromes result from mutations at the same nucleotide (m.8993T>G or, less frequently, m.8993T>C) in the gene encoding the ATPase 6 subunit of complex V. Patients with NARP typically are young adults with the acronymic features whereas maternally related children are likely to have the characteristic symptoms and signs of LS, with developmental delay or arrest, respiratory abnormalities, frequent vomiting, nystagmus, pyramidal signs, bilateral lesions in the basal ganglia and the brainstem, and lactic acidosis ( Figure 41.6A,B , Leigh synd). Retinitis pigmentosa distinguishes MILS from other forms of LS and is a useful diagnostic clue. We now know that the different age at onset and severity of NARP and MILS depend on the mutation load: patients with NARP harbor ~70% mutant mtDNAs whereas patients with MILS harbor ~90%.
Leber’s Hereditary Optic Neuropathy
Leber’s hereditary optic neuropathy (LHON) is the most common of the mtDNA-based diseases and the first disorder attributed to a mtDNA point mutation. It causes subacute loss of central vision in young adults, predominantly men. LHON is usually due to homoplasmic mutations in one of three genes encoding complex I subunits (m.11778G>A in ND4; m.3460G>A in ND1, or m. 14484T>C in ND6). Despite the homoplasmic nature of these mutations, the retinal ganglion cells are selectively affected and myopathy is not a feature. The predominant involvement of men has been attributed to a protective effect of estrogens in women, an epigenetic effect on the expression of a mtDNA-based disease.
Isolated Myopathies
As of 2000, there were 14 cases of isolated myopathy, with exercise intolerance, weakness, and—often—recurrent episodes of rhabdomyolysis and myoglobinuria associated with mutations in subunits of complex I, III, or IV (Table 3 in ). Most of the mutations were in the gene ( MTCYB ) encoding cytochrome b (see Case Example 41.2 ) and all of them were de novo somatic changes in sporadic patients. Most patients were adult, although in some of them exercise intolerance had started in childhood. Severe exercise intolerance with or without myoglobinuria and without evidence of maternal inheritance justifies a muscle biopsy, especially if blood lactate is elevated.
A 52-year-old woman had had exercise intolerance since childhood. She remembered rushing to a friend’s house at the age of 5 years and arriving there “exhausted and nauseous.” These symptoms progressed until she was unable to walk more than one block without experiencing extreme fatigue. She also noted premature fatigue and aching of masticatory muscles when chewing tough meat. Examination at age 39 revealed weakness against resistance in proximal limb muscle. Resting venous lactate concentration was 5 mg/deciliter (normal <2.2). She never noted pigmenturia. Standardized bicycle ergometry with 31 P-nuclear magnetic resonance spectroscopy showed a low ratio of phosphocreatine to inorganic phosphate (PCr/Pi) at rest. The ratio decreased further with exercise and, after cessation of exercise, returned to baseline more slowly than normal. Electromyography of limb muscle showed short-duration motor units and polyphasic potentials, suggesting mild myopathy. There was no family history of neuromuscular diseases: the patient’s mother had died of cancer at age 69 years and her brother and two children were in good health. Although she reported shortness of breath with exercise, her cardiac function was normal. Serum creatine kinase (CK) concentration was normal, but resting serum lactate concentrations were elevated. A muscle biopsy at age 39 revealed numerous COX-positive RRF and biochemical analysis showed marked isolated complex III deficiency. Sequencing of the gene ( MTCYB ) encoding cytochrome b identified a heteroplasmic m14846G>A mutation (p.G34S). Single fiber analysis showed that the mutation was much more abundant in RRF than in normal fibers.
Comment
This patient, as well as four others reported by Andreu et al., was not considered to have a mtDNA mutation because she had a tissue-specific (as opposed to a multisystemic) disorder, no evidence of maternal inheritance, and an isolated biochemical defect (complex III deficiency). In fact, she is typical of mutations in protein-coding genes of mtDNA and the diagnosis—suggested by extreme exercise intolerance and resting hyperlactacidemia—was confirmed by the muscle biopsy showing COX-positive RRF. The sporadic nature of these myopathies is due to de novo somatic mutation affecting exclusively myogenic stem cells after germ-layer differentiation.
Other Presentations
The most common clinical presentations associated with mtDNA mutations in protein-coding genes have been MELAS and LS. Both conditions have been attributed to the m.13513G>A mutation in MTND5 while LS has also been associated with diverse mutations in MTND3 . We noted that patients with LS and the common m.10191T>C mutation in MTND3 tend to have unusually long survival.
Diseases Due to Nuclear DNA Mutations
In the course of evolution, mitochondria have lost much of their bacterial independence and now rely on the nucleus for most of their functions. Therefore, Mendelian mitochondrial disorders are numerous and varied. We can discern at least six disease categories because pathogenic mutations can affect: (1) genes encoding subunits of the respiratory chain (direct hits); (2) genes encoding assembly proteins of respiratory chain complexes (indirect hits); (3) genes needed for mtDNA translation; (4) genes controlling the phospholipid composition of the mitochondrial inner membrane (MIM); (5) genes involved in mitochondrial dynamics. And, as discussed previously, we will consider separately the sixth category, i.e. defects in genes needed for mtDNA integrity or replication (“mtDNA maintenance”).
The advent of whole exome sequencing and of the more narrowly targeted mito-exome sequencing has allowed us to sequence in one sweep the approximately 1700 nuclear genes encoding mitochondrial proteins. Mitoexome sequencing has already enriched all six disease categories with new variants, and it is to be expected that it may reveal novel disease categories.
Direct Hits
These disorders result from nDNA mutations affecting each of the five respiratory chain complexes. We will review them following the conventional sequential order of the complexes in the respiratory chain but it should be kept in mind that defects in one complex may well have a “ripple effect” because of the supercomplex organization of the respiratory chain.
Complex I
Complex I (NADH-ubiquinone oxidoreductase) is the Goliath of the respiratory chain, containing 45 subunits, of which 38 are encoded by nDNA. Given its size, it is not too surprising that isolated complex I deficiency is the most frequently encountered respiratory chain defect.
Pathogenic mutations have been identified in all of the 14 evolutionarily conserved subunits that comprise the catalytic core. The resulting clinical pictures are remarkably homogeneous and largely correspond to LS. LS is defined neuropathologically (or neuroradiologically) by bilateral symmetrical lesions all along the nervous system, but especially in the basal ganglia, thalamus, brainstem, and cerebellar roof nuclei. Microscopically, there is neuronal loss, proportionate loss of myelin, reactive astrocytosis, and proliferation of cerebral microvessels.
Clinically, these children have psychomotor retardation or regression, respiratory abnormalities, hypotonia, failure to thrive, seizures, dystonia, nystagmus, and optic atrophy. These are the symptoms most commonly encountered by Distelmaier et al. in a study of 15 children with complex I deficiency due to mutations in nDNA-encoded genes, and their findings were reinforced by a review of 130 patients reported in the literature.
Besides LS, four distinct presentations had been attributed to Mendelian complex I deficiency: fatal infantile lactic acidosis (FILA), neonatal cardiomyopathy with lactic acidosis, leukodystrophy with macrocephaly, and hepatopathy with renal tubulopathy. However, no specific genotype/phenotype emerged for the different variants and it is more likely that differences in residual activity may account for the apparent differential tissue involvement.
One important clinical feature common to these disorders is that onset is invariably in infancy or childhood but almost never in fetal life. Usually, these children are fine at birth and early in life, although onset of symptoms is followed by a rapid and relentless progression leading to early death. Lactic acid is consistently and markedly elevated both in blood and in the CSF. From a genetic point of view, it is noteworthy that mutations in NDUFA1 cause X-linked LS, a most unusual hereditary pattern for respiratory chain defects.
Complex II
Complex II (succinate-ubiquinone oxidoreductase) is the smallest multimeric component of the respiratory chain, comprising only 4 subunits. Complex II deficiency is also probably the least common respiratory chain defect. Although only a handful of patients have been described, clinical presentations varied and muscle weakness was usually a consequence of central nervous system involvement.
Four patients had typical LS. Of these, two children had psychomotor regression and spastic quadriparesis with diffuse leukodystrophy by MRI, and a third child presented in the first year of life with stunted growth and lactic acidosis without neurological symptoms. Interestingly, all three children stabilized or improved after riboflavin administration.
Complex III
Two distinct direct hits for complex III (ubiquinol-cytochrome c reductase) have been described, one in a single patient and the other in a large consanguineous family. A deletion in the UQCRB gene encoding the ubiquinone-binding protein (QP subunit or subunit VII) was identified in an 8-month-old girl with moderate hepatomegaly, hypoglycemia, metabolic acidosis, and lactic acidosis. At the age of 4 years, the child was normal and the hepatomegaly had regressed. Complex III activity was decreased in liver, lymphocytes, and fibroblasts.
Twenty members of an Israeli-Bedouin family presented with a uniform encephalomyopathy that was severe but compatible with long survival. These patients were normal at birth and for the first few months, then showed psychomotor retardation and developed extrapyramidal signs, including dystonia, athetoid movements, ataxia, and dementia. There was a homozygous mutation in UQCRQ .
Coenzyme Q10 Deficiencies
This relatively new group of disorders due to deficiency of Coenzyme Q10 (CoQ10) (ubiquinone) can arguably be included in the “direct hits” because mutations in a cascade of biosynthetic enzymes results in the deficiency of one relatively simple component of the respiratory chain ( Figure 41.3 ). CoQ10 shuttles electrons from complex I and complex II to complex III, acts as an antioxidant, and regulates apoptosis. CoQ10 deficiency was first described in 1989 in two sisters with the clinical triad of mitochondrial and lipid storage myopathy, recurrent muscle breakdown with myoglobinuria, and encephalopathy with seizures, ataxia, and mental retardation. CoQ10 deficiency was also noted in children with spinocerebellar atrophy-like presentations, but molecular defects in biosynthetic genes ( PDSS2 and COQ2 ) were not reported until 2006 in two infants with severe infantile mitochondrial encephalomyopathies and glomerular renal involvement. Mutations in the same and other biosynthetic genes ( PDSS1 , COQ6 , ADCK3 , and COQ9 ) followed and five clinical syndromes are now attributed to CoQ10 deficiency: (i) encephalomyopathy; (ii) cerebellar ataxia; (iii) infantile multisystem disorder; (iv) isolated nephropathy; (v) isolated myopathy. It is important to consider primary CoQ10 deficiency in the differential diagnosis of juvenile ataxias and infantile encephalomyopathies because most patients with these disorders benefit from CoQ10 supplementation. (See Case Example 41.3 .)
A 33-month-old boy, the son of first cousins, had hypotonia, mild psychomotor delay, and optic atrophy. At 12 months, proteinuria led to a renal biopsy, which showed focal and segmental glomerulosclerosis. His younger sister also had proteinuria. At 18 months, he developed frequent vomiting requiring peritoneal dialysis. He then developed psychomotor regression (loss of the ability to walk or stand unassisted), tremor, and new-onset status epilepticus with focal EEG abnormalities predominant in the left occipital region. Brain MRI showed mild cerebellar atrophy and stroke-like lesions in the left cingulate cortex and subcortical area. Blood and CSF lactate levels were normal. At 22 months, he developed right hemiplegia, myoclonus, and swallowing difficulties. A muscle biopsy showed that many fibers showed increased SDH staining, although they were not ragged-red or COX-deficient. Biochemical analysis of muscle homogenate revealed decreased activities of complex I+III, whereas other complexes were normal. The concentration of CoQ10 was markedly decreased in skeletal muscle (12 μg/g fresh tissue; normal, 32.1±6.7) and in fibroblasts (19 ng/mg protein; normal, 105±14). With oral CoQ10 supplementation (30 mg/kg/day), all neurological manifestations improved dramatically whereas his renal function did not improve and at age 3 years he needed a renal transplantation. He is now 7 years old and his kidney-allograft is functioning normally, but he has neurological sequelae, including cognitive impairment, seizures, and hemiplegia. His sister, who was diagnosed with CoQ10 deficiency and nephrosis at 1 year, before any neurological symptoms developed, was treated with oral CoQ10 and her nephrotic syndrome slowly resolved. Homozygosity mapping followed by candidate gene sequencing revealed a homozygous mutation in COQ2 , the gene encoding para -hydroxybenzoate-polyprenyl transferase, the CoQ10 biosynthetic enzyme that catalyzes the conjugation of the benzoquinone ring with the decaprenyl side chain.
Comment
This child had a severe infantile mitochondrial encephalomyopathy and nephrosis due to primary CoQ10 deficiency and was the first patient in whom a molecular defect of CoQ10 biosynthesis was documented by Quinzii et al. This case is a reminder that the association of infantile encephalomyopathy and nephrosis should raise the suspicion of primary CoQ10 deficiency, which can be documented in skeletal muscle or in cultured skin fibroblasts. These two siblings also remind us that most forms of primary CoQ10 deficiency respond to oral CoQ10 supplementation, albeit not always completely.
Complex IV
Probably most “direct hits” in nuclear encoded subunits of complex IV (COX) are incompatible with life because years of research have revealed mutations in only one of the 10 nDNA-encoded subunits, COX6B1. Although a sister of the patients had died immediately after birth and the mother had had a second trimester miscarriage, of the two affected brothers one had died at 10 years of age and the other was 8 years old at the time of publication. Both children had had failure to thrive but normal psychomotor development until late childhood, when they developed muscle weakness, cognitive deterioration, visual problems, and lactic acidosis. MRI showed severe cavitating leukodystrophy in both.
Complex V
“Direct hits” of complex V also appear to be very rare, having been reported only in one patient, who harbored a homozygous mutation in the ATP5E gene. This girl (patient 3 in ), was small at birth and had poor suck, respiratory distress, lactic acidosis, and 3-methylglutaconic aciduria. Although she had recurrent metabolic crises, her clinical course stabilized at 5–6 years and she completed school and was gainfully employed. At the age of 17 years, she had mild ataxia, horizontal nystagmus, exercise intolerance, mixed axonal and demyelinating polyneuropathy, and mild left ventricular hypertrophy. Brain MRI had shown hyperintense lesions in the caudate and lentiform nuclei at age 14, but had normalized by age 17.
Indirect Hits
Even correctly encoded subunits of the various complexes have to be translated, imported into mitochondria, and directed to the MIM. Here, they must assemble with their mtDNA- and nDNA-encoded counterparts and acquire prosthetic groups; some of them multimerize; and most of them must further assemble into supercomplexes. Mutations in genes controlling these pathways are called “indirect hits” because they affect the respiratory chain indirectly.
Complex I
Pathogenic mutations have been reported in 6 assembly subunits and in 5 chaperone factors. It is safe to predict that many more mutated genes in both groups will be identified through the increased use of whole exome sequencing. (See Case Example 41.4 .)
A 13-year-old boy came to medical attention because of exercise intolerance, weakness, and muscle wasting. He was born after normal pregnancy and delivery but showed psychomotor delay since the first year of life. Neurological examination showed proximal muscle weakness, generalized hypotonia, ataxic gait, bradykinesia and bradylalia, scoliosis, and truncal obesity. A cognitive assessment revealed mild deficit (his IQ was 81). Cardiac function was normal as were both brain MRI and MRS. Laboratory tests showed increased resting plasma lactate and alanine and increased urinary lactate. He also had hypothyroidism. Electromyography was compatible with a myopathic process and a muscle biopsy showed excessive subsarcolemmal staining with the Gomori trichrome stain and with the COX reaction, indicating mitochondrial proliferation. Biochemical analysis of a muscle homogenate showed severe complex I deficiency and moderate complex III deficiency, whereas complex II and citrate synthase values were above normal. Next-generation exome sequencing using a mitochondrial gene library (mito-exome sequencing) revealed a homozygous mutation (c.1240C>T) in the ACAD gene, predicted to be deleterious. The patient was treated with riboflavin. He is now 19 years old and has nonprogressive exercise intolerance and weakness.
Comment
This patient, recently reported by Garone et al., had a mild and rather nonspecific mitochondrial encephalomyopathy with complex I (and less severe complex III) deficiency. Because the trait appeared to be autosomal recessive, we resorted to mito-exome sequencing, which revealed a new mutation in a complex I assembly gene. Mutations in ACAD9 had been associated with severe and rapidly fatal hypertrophic cardiomyopathy of infancy but also with milder childhood-onset myalgia and exercise intolerance. The beneficial effect of riboflavin, a catalytic cofactor of the ACAD proteins, was documented in cultured skin fibroblasts from the patients with cardiopathy and clinically in the patients with exercise intolerance. This case exemplifies an “indirect hit” to complex I and illustrates the power of whole or mitochondrial exome sequencing in the identification of clinically undefined mitochondrial disorders.
The clinical manifestations tend to be more heterogeneous than those associated with “direct hits” in complex I, although there is a good deal of overlap. All the patients had encephalopathy clinically resembling LS but often with leukodystrophy rather than gray matter involvement. Cardiomyopathy was more common and sometimes the dominant feature. Although onset was in infancy or early childhood and early death was common, the course was often prolonged and in some cases fluctuating. Death occurred between 5 and 13 years of age in three patients and a few patients were alive at ages 4, 5, and 20 years at the times of publication.
Three siblings in a doubly consanguineous Dutch family and two unrelated patients, who harbored mutations in the ACAD9 gene, had isolated myopathy with exercise intolerance and lactic acidosis responsive to riboflavin.
Complex II
Even the small complex II requires assembly factors; and mutations have been described in one of them ( SDHAF1 ), which contains a LYR motif and is presumably involved in Fe-S metabolism. After developing normally for the first 6 to 11 months, five children from two consanguineous families developed acute psychomotor regression, impaired growth, spastic quadriplegia, and moderate cognitive decline. One child died at 18 months but the others were alive at ages 6 to 12 years at the time of publication. MRI and positron MRS of the brain showed leukoencephalopathy, sparing U fibers and increased lactate and succinate peaks.
In 1991 and 1993, Haller and coworkers described a young Swedish man with lifelong exercise intolerance, dyspnea, cardiac palpitations, and episodes of myoglobinuria. The syndrome was called “mitochondrial myopathy with succinate dehydrogenase and aconitase deficiency” and attributed to altered metabolism of iron-sulfur (Fe-S) cluster proteins, which are prosthetic groups present in complexes I, II, III, and in the Krebs cycle enzyme aconitase. Accordingly, histochemical analysis of muscle showed SDH deficiency, and biochemical analysis showed deficiencies of complex II, complex III, and aconitase. In 2008, homozygosity mapping revealed a single pathogenic mutation in the ISCU gene in three Swedish families, and two years later, Kollberg et al. described SDH deficiency and accumulation of iron in muscle as the morphological hallmarks of the disease.
A combined defect of complex II and III in muscle was caused by a homozygous mutation in the IBA57 gene, which encodes yet another assembly factor for mitochondrial [4Fe-4S] cluster proteins. Clinically, this condition in two siblings was characterized by hypotonia, respiratory insufficiency, arthrogryposis, microcephaly, congenital brain malformations, hyperglycinemia, and perinatal death.
Complex III
The first assembly defect in complex III was identified in 2002 in Finnish infants with an extremely severe syndrome named GRACILE, which summarizes the main symptoms and signs: growth retardation, aminoaciduria, cholestasis, iron overload, and early death (before 5 months of age). The mutated protein, BCS1L, is an ATPase needed for insertion of the Rieske FeS subunit into the complex. Interestingly, these children did not have neurological impairment nor overt complex III deficiency. In contrast, British patients with GRACILE syndrome had both neurological involvement (hypotonia, seizures) and decreased enzyme activity. In even sharper contrast, five Turkish children from four unrelated but consanguineous families developed severe hepatopathy (with complex III deficiency in liver) soon after birth, proximal renal tubulopathy, and encephalopathy compatible with LS in two cases.
Two unrelated patients with mutations in BC1IL suffered from severe encephalopathy without visceral involvement: both showed respiratory distress at birth and developed psychomotor delay or regression, spastic quadriparesis, and seizures in one case. Both patients had cerebral atrophy by MRI and, curiously, both had brittle hair. One patient with a novel homozygous BC1IL mutation had a mild presentation with infantile onset of hypotonia, psychomotor retardation, coarse facial features, and hirsutism.
The most benign expression of mutations in BCS1L is Björnstad syndrome, characterized by congenital sensorineural hearing loss and pili torti, resulting in brittle hair, usually recognized in childhood.
A second assembly factor for complex III is tetratricopeptide repeat 19 (TTC19), a protein of the MIM, where it interacts with complex III. Four Italian patients with homozygous mutations in TTC19 had severe encephalopathy. Two siblings presented in childhood with neurological symptoms that progressed for years and included mental retardation, ataxia, nystagmus, dysphagia, dysphonia, tremor, dystonia, and hearing loss. The sister was alive but confined to a wheelchair at age 30 and her younger brother was totally incapacitated at age 20. A third patient also had childhood-onset mental retardation, dystonia, and ataxia. At age 19, she was bedridden, on a ventilator, and in a fluctuating comatose state. The fourth patient did not seek medical attention until 42 years of age, when he developed limb weakness and fasciculations, apraxia of gait and hand movements, dysarthria, dystonia, and paraparesis. He died at age 45.
Complex IV
It had long been known that COX (complex IV) deficiency is a common cause of LS, but years went by before a mutated gene was associated with LS: the gene ( SURF1 ) is essential for correct COX assembly and this was the first example of an “indirect hit” to affect the respiratory chain. By 2001, 30 different mutations had been identified.
Mutations in the COX assembly gene SCO2 , which encodes a metallochaperone involved in mitochondrial copper delivery, cause a much more severe clinical phenotype that combines neonatal hypertrophic cardiomyopathy with encephalopathy, is fatal in the first weeks or months of life, and may be associated with early fetal lethality. The fatal infantile presentation is typical of compound heterozygous patients, who always harbor the “common” E140K mutation. Homozygosity for the same mutation causes delayed onset and longer survival.
Importantly, SCO2 mutations (both in compound heterozygosity and in homozygosity) can simulate spinal muscular atrophy (SMA) and the neurogenic, often SMA-like, histological pattern of the muscle biopsy further complicates the differential diagnosis. One postmortem study of the spinal cord showed severe neuronal loss and astrocytosis in the anterior horns. Two distinctive features orient towards the correct diagnosis: clinically, the severe cardiomyopathy; and, morphologically, the lack of COX stain in the muscle biopsy. However, in patients with the clinical picture of SMA but without mutations in the SMN1 gene, it is important to consider SCO2 mutations.
Mutations in SCO1 , a second metallochaperone needed for copper insertion into the COX holocomplex, were first published on in 2000 and reported to affect multiple members of a large family. The child described in that paper was hypotonic and lethargic at birth, had severe metabolic acidosis, and needed respiratory assistance. Liver function tests were severely altered at day 4, followed by hepatomegaly. He developed axial hypotonia, episodes of apnea and bradycardia, and died at 2 months. A liver biopsy obtained immediately postmortem showed swollen hepatocytes with microvesicular steatosis, and a muscle biopsy showed lipid storage. A sibling presented with metabolic acidosis and “severe neurological distress” and died at 5 days.
The report of a single family suggests that SCO1 mutations are either incompatible with life or very rare, a conclusion supported by several genetic screenings of patients with COX deficiency.
Mutations in COX10 , which encodes a factor involved in the first step of the mitochondrial heme-A biosynthesis, were described in five patients from three families and with different clinical presentations. Three siblings from a consanguineous family died at 2, 3, and 5 years of age: the child described in more detail was normal until 18 months, when he developed ataxia, weakness, ptosis, pyramidal signs, and status epilepticus. He had lactic acidosis and increased urinary amino acids, suggesting proximal renal tubulopathy. His younger sister was reported to have similar “progressive neurological deterioration.” One of two unrelated children had lactic acidosis, hypotonia, growth retardation, sensorineural hearing loss, transfusion-dependent macrocytic anemia, and biventricular hypertrophic cardiomyopathy, and died at 5 months. The other child had a sudden arrest of her psychomotor development at one and a half months, and developed transfusion-dependent anemia, mild splenomegaly, and hypotonia. Brain MRI showed symmetrical lesions in the basal ganglia typical of LS. She died of central respiratory failure at 4 months.
COX15, like COX10, is involved in heme-A biosynthesis and mutations in COX15 caused hypotonia, seizures, and lactic acidosis in an infant girl, who had a normal echocardiography at 6 days of age but developed acute biventricular hypertrophy by 22 days and died 2 days later. Postmortem examination revealed massive accumulation of morphologically abnormal mitochondria in the heart.
The heart was not affected in a second patient, who presented with typical LS: he was normal at birth and for the first seven months, then developed hypotonia, nystagmus, leg spasticity, progressive microcephaly, and retinopathy. He died of pneumonia a year later. Lactic acid was increased both in blood and in the CSF and brain MRI showed multiple symmetrical lesions in the basal ganglia, dorsal midbrain, cerebral peduncles, and periaqueductal gray matter. A third patient also had a diagnosis of LS, but her course was unusually protracted and, at the time of publication, she was still alive at age 16.
Considering that at least 30 chaperones are needed for the assembly of complex IV in yeast, it is not surprising that the list of indirect hits responsible for COX deficiency in humans keeps getting longer. On the other hand, it is difficult to make general statements on clinical phenotypes because mutations in some genes have been reported in single or very few patients, often siblings from consanguineous marriages. This is the case for a homozygous mutation in COA5 , which encodes a factor involved in the early steps of COX assembly. Two siblings had onset of cardiomyopathy in utero with fetal distress and isolated biventricular hypertrophic cardiomyopathy causing death 8 and 10 days after birth. Postmortem examination of the heart revealed mitochondrial proliferation and lipid storage.
Two siblings from another consanguineous family had a slower but devastating disorder dominated by encephalopathy, with severely delayed psychomotor development, optic atrophy, intractable seizures leading to status epilepticus in the affected boy, and left hemiplegia in the affected girl. At 14 years of age, the girl was unable to stand or walk and had a 20-word vocabulary. The boy was lost to follow-up at the age of 4 years, when he was bedridden and incapable of communicating or moving purposefully. The mutant gene ( KIAA0971 ), identified through homozygosity mapping, encodes a protein known as fas-activated serine-threonine kinase domain 2 (FASTKD2) that plays a role in mitochondrial apoptosis rather than in COX assembly. Although COX activity was markedly decreased in muscle, COX and SDH histochemical stains were normal.
Through integrated genomics, based on bioinformatics-generated intersection of DNA, mRNA, and protein data sets, Mootha et al. identified the gene ( LRPPRC ) responsible for the French Canadian type of LS (LSFC) with COX deficiency. Because the LRPPRC (leucine-rich pentatricopeptide repeat cassette) protein has a role in the translation or stability of the mRNA of mtDNA-encoded COX subunits, LSFC might be more appropriately classified among the defects of mtDNA translation (see the following).
Another defect of mitochondrial translation affecting COX, and specifically the COXI subunit, is caused by mutations in the gene TACO1 (translational activator of COXI). Five children of a consanguineous Turkish family were affected with slowly progressive LS, more severe in girls than in boys. Onset was in childhood or adolescence and progression was slow: all patients were alive at the time of publication and three were in their 20s. The clinical picture was dominated by small stature, mental retardation, dystonia, dysarthria, spasticity with pyramidal signs, and optic atrophy. Lactic acid was inconsistently increased, and MRI of the brain showed bilateral basal ganglia lesions in all patients.
Integrative genomics also facilitated identification of the ETHE1 gene in children with ethylmalonic encephalomyopathy (EE), an early-onset disorder with microangiopathy, chronic diarrhea, and greatly increased levels of ethylmalonic acid and short-chain acylcarnitines in body fluids. Based on their studies of patients and Ethe1 -null mice, Valeria Tiranti and Massimo Zeviani introduced a new paradigm of “indirect hit,” which can be called “toxic indirect hit.” They documented that ETHE1 is a matrix thioesterase and its dysfunction leads to excessive accumulation of sulfide, a powerful COX inhibitor. The clinical presentation has been confirmed and the number of mutations extended in a study of 14 patients with EE.
Complex V
Mutations in two ancillary proteins cause severe infantile disorders. A homozygous missense mutation in ATP12 (now known as ATPAF2 ) was first reported in 2004 in an infant girl who died at 14 months. She had dysmorphic features, arthrogryposis, hepatomegaly, hypoplastic kidneys, fluctuating lactic acidosis, and—terminally—seizures. Brain MRI showed cortical-subcortical atrophy, dysgenesis of the corpus callosum, and hypoplastic white matter.
A homozygous mutation (c.317-2A>G) in the assembly gene TMEM70 was identified in 25 infants, of whom 24 were of Roma ethnic origin, with severe multisystem symptoms, lactic acidosis, and 3-methylglutaconic aciduria. The clinical picture was described in detail in a subsequent paper and was characterized by neonatal hypotonia, apneic spells, hypertrophic cardiomyopathy, severe lactic acidosis, and hyperammonemia. Ten patients died within 2 months and the survivors showed hypotonia and psychomotor delay. The cardiomyopathy was nonprogressive and sometimes reversible. The authors suggest that mutations in TMEM70 should be suspected in critically ill neonates with hypotonia, hypertrophic cardiomyopathy, and the laboratory triad of lactic acidosis, hyperammonemia, and 3-methylglutaric aciduria. The report of more patients has widened the clinical spectrum, including congenital hypertonia with multiple contractures, early-onset cataracts and gastrointestinal problems, and Reye syndrome-like episodes.
Defects of Mitochondrial Protein Importation
A prerequisite for the assembly of any respiratory chain complex is the import of nDNA-encoded subunits from the cytoplasm into mitochondria. Surprisingly, considering the complexity of the mitochondrial importation machinery, only a few diseases belong to this category.
One is the Mohr-Tranebjaerg deafness-dystonia syndrome (DDS), due to mutations in the MIM protein DDP1 (deafness dystonia protein 1) encoded by the gene DDP/Tim8 . Besides sensorineural deafness and dystonia, patients suffer from spasticity, dysphagia, cognitive deterioration, and cortical blindness.
Mutations in the chaperonin Hsp60 cause an autosomal dominant form of hereditary spastic paraplegia, HSP13, whereas mutations in another chaperonin, DNAJC19, cause early-onset dilated noncompaction cardiomyopathy, anemia, ataxia, testicular dysgenesis, and 3-methylglutaconic aciduria.
The GFER gene encodes a sulfydryl oxidase (DRS) of the mitochondrial intermembrane space, which is involved in mitochondrial protein import. A homozygous mutation of GFER caused congenital cataract, progressive muscle hypotonia, sensorineural hearing loss, and developmental delay in three siblings of a consanguineous family. The pathogenic relationship between this gene and the multiple mtDNA deletions observed in muscle biopsies is not clear.
Defects of mtDNA Translation
Clinically, these disorders came to the attention of pediatricians because they cause severe infantile syndromes characterized by neurological involvement (LS, leukodystrophy, recessive ataxia), hepatocerebral syndrome, or cardiomyopathy, similar to those associated with mtDNA depletion even though mtDNA is normal both qualitatively and quantitatively. Although these disorders are usually characterized by multiple respiratory chain enzyme deficiencies, mutations in some translation factors, such as LRPPRC and TACO1, impair specifically COX activity, as described previously.
Translation of mitochondrial mRNAs into the 13 mtDNA-encoded respiratory chain subunits comprises four phases, each requiring multiple ancillary factors, including initiation factors (IF2 and IF3), elongation factors (EF-Tu, EF-Ts, and EF-G1), release factors (eRF1 and ICT1), translational activators (TACO1 and LRP130), and specific tRNA base modifiers (TRMU and PUS1).
Examples of specific disorders due to defective mtDNA translation are given in recent reviews. We have already described the reversible infantile COX-deficient myopathy due to the combination of a mtDNA tRNA Glu homoplasmic mutation and downregulation of the tRNA Glu base modifier TRMU. Another defect of mtDNA translation with prominent neuromuscular involvement is MLASA (myopathy, lactic acidosis, sideroblastic anemia). This autosomal recessive disorder causes weakness and anemia in childhood and may lead to dependency on blood transfusion. Although the selective vulnerability of skeletal muscle and bone marrow remains difficult to explain, one cause of the disease is deficient pseudouridylation of mitochondrial tRNAs due to mutations in the gene ( PUS1 ) encoding pseudouridylate synthase 1. A second cause of MLASA is a defect of tyrosyl-tRNA-synthetase due to mutations in the gene YARS2 .
Yet another disease of muscle and the bone marrow that combines mitochondrial myopathy with megaloblastic anemia was recently described in two children and attributed to mutations in the gene SFAX4 , which encodes sideroflexin 4, a MIM transmembrane protein, probably an iron transporter. As the biochemical defect in these cases involved complex I, this disorder may more appropriately belong to the “indirect hits” (see above).
Defects of the Mitochondrial Inner Membrane Lipid Milieu
The phospholipid component of the mitochondrial inner membrane (MIM) is not just a convenient scaffold for the respiratory chain but it is also an integral functional partner. Increasingly, alterations of the lipid milieu are being associated with mitochondrial encephalomyopathies and—importantly—many of them are neuromuscular disorders.
The first example of these disorders is Barth syndrome, an X-linked myopathy and cardiopathy, with neutropenia and impaired growth. Barth syndrome is due to mutations in the tafazzin ( TAZ ) gene, resulting in deficiency or abnormal composition of cardiolipin, the major phospholipid component of the MIM.
In the past few years, four new entities have been described, affecting different phospholipids. Sengers syndrome is similar to Barth syndrome in that: (i) both conditions were described by Dutch pediatricians; (ii) they are both characterized by mitochondrial myopathy and cardiopathy, although in Sengers syndrome congenital cataracts are a distinctive additional sign; and (iii) cardiolipin alterations are involved in both conditions, although in Sengers syndrome they are secondary to mutations in the acylglycerol kinase ( AGK ) gene. The resulting decrease of phosphatidic acid and lysophosphatidic acid in the MIM presumably explains the lack of the adenine nucleotide translocator 1 (ANT1), which was initially considered causative of the disease. The typical clinical triad of myopathy, cardiopathy, and congenital cataracts was further complicated by vascular strokes in one of two families with molecularly documented Sengers syndrome. Furthermore, these patients had extremely high citrate synthase (CS) activity in muscle and CS crystals were identified in muscle mitochondria.
A human congenital muscular dystrophy with giant mitochondria (a megaconial myopathy, according to Shy and Gonatas ) displaced to the periphery of the muscle fibers was attributed to mutations in the gene ( CHKB ) encoding choline kinase beta when it was realized that clinical picture and muscle morphology were similar to those of a spontaneously mutant mouse strain with rostrocaudal muscular dystrophy. (See Case Example 41.5 .) The human congenital myopathy is compatible with prolonged survival, but all patients have, besides delayed motor development, severe intellectual disability with autistic features and may develop dilated cardiomyopathy.
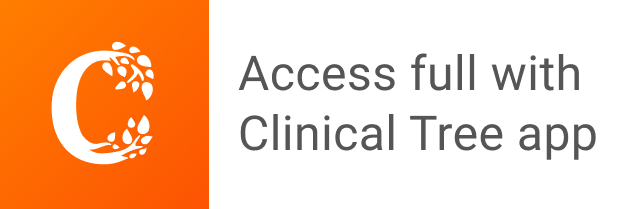