Chapter 103 Genetics of Obstructive Sleep Apnea
Abstract
Definition of the Obstructive Sleep Apnea–Hypopnea Phenotype
Most family and genetic studies of OSAH have used the apnea–hypopnea index (AHI) to define phenotype. The advantages of using the AHI include its relative simplicity, moderate to high night-to-night reproducibility,1 and widespread clinical use. In addition, it is often used clinically to diagnose cases and to justify third-party reimbursement for treatment, and it is often followed as a key outcome in OSAH treatment studies. Because the AHI has been shown to be moderately correlated with other indices of OSAH severity, such as nighttime oxygen desaturation and sleep fragmentation, it may provide information about several correlated traits that are important in disease expression. All genetic studies of OSAH that use the AHI as the outcome variable have demonstrated significant familial aggregation, suggesting that this measure captures useful information for quantifying genetic associations.
Combining polysomnographic data with other information, including symptoms, signs, and outcome data, to derive a multidimensional phenotype is one approach for producing a more comprehensive description. In the Cleveland Family Study, a stronger relationship between familial risk and OSAH was observed when OSAH was defined by an AHI level greater than 15 plus reported daytime sleepiness than when disease was defined by AHI level alone.2 Additional power may be gained in future genetic studies of OSAH that use multidimensional phenotypes. As part of this effort, the polysomnographic variables that best identify specific phenotypes must be clarified. For example, alternatives to the AHI, such as indices of flow limitation during sleep or spectral analysis of sleep architecture, may prove to be superior markers for genetic studies. However, a phenotype must be feasible for use in the large numbers of subjects who are needed for genetic epidemiologic studies of complex traits. The choice of phenotype will be influenced by the cost, degree of invasiveness, and individual burden required for identifying and quantifying the phenotype and for applicability across the spectrum of age and body mass index (BMI), as well as by its accuracy and reliability.
Intermediate Phenotypes
A number of risk factors probably interact to increase propensity for repetitive upper airway collapse that occurs during sleep in patients with OSAH. In a given person, the relevant attributes may be determined by anatomic and neuromuscular factors that influence upper airway size and function. Strong OSAH risk factors are obesity and male gender. Although it has been argued that the genetics of obesity cannot be separated from the genetics of OSAH, careful statistical modeling of AHI and BMI indicates that only about 35% of the genetic variance in AHI is shared with BMI, suggesting that a substantial portion of the genetic basis for OSAH is independent of obesity.3 Other pathoetiologic pathways include those that influence upper airway size, ventilatory control mechanisms, and possibly elements of sleep and circadian rhythm control.
Thus, it is useful to consider at least four primary intermediate pathogenic pathways through which genes might act to increase susceptibility to OSAH: obesity and related metabolic syndrome phenotypes, craniofacial and upper airway morphometry, control of ventilation, and control of sleep and circadian rhythm4; these are discussed in more detail later. The limitation of this approach is that the genes so identified might not be sufficient to describe the clinically important phenotype, which might only occur in the context of other genetic and environmental factors. Specifically, susceptibility genes for intermediate traits associated with OSAH might not be equivalent to the susceptibility genes for OSAH.
Obesity and Body Fat Distribution
Obesity increases risk of OSAH by 2- to 10-fold, with the strongest associations observed in middle-age.5 There are several pathways through which obesity predisposes to OSAH. Fat deposition in the parapharyngeal fat pads may directly narrow the upper airway and predispose it to collapse when neuromuscular activation of upper airway muscles declines with sleep (see Chapter 101). Fat deposition in the thorax and abdomen also increases the work of breathing, which can produce hypoventilation and reduce lung volumes, which in turn reduces parenchymal traction on the trachea, making the airway more collapsible. Reduced lung volumes also can increase propensity for oxygen desaturation to occur, increasing the likelihood that any given reduction in airflow may be classified as a hypopnea and increasing the severity of hypopnea-associated physiologic disturbances. In addition, low lung volumes can reduce oxygen stores and alter loop gain, which, in turn, can influence ventilatory instability. Finally, adipose tissue secretes hormones such as leptin that can influence ventilatory drive (see later). Central body fat distribution appears especially important in the pathogenesis of OSAH. It is unclear whether this is because of the mechanical effects of central fat on lung mechanics and ventilation or upper airway size or because visceral fat is metabolically active.
Heritability estimates for obesity-associated phenotypes such as BMI, skinfold thickness, regional body fat distribution, fat mass, and leptin levels range between 40% and 70%, consistent with moderate to strong influences of genetic factors on these traits.6–8 Approximately 7% of cases of early-onset obesity have been estimated to be attributable to the effects of mutations in at least seven genes influencing the leptin–melanocortin pathways, including melanocortin-4 receptor, leptin, leptin receptor, proopiomelanocortin, and tropomyosin-related kinase-B, which largely influence weight though alterations in appetite regulation.9 Mutations in melanocortin-4 receptor increase risk of severe childhood obesity by approximately 30% and also have been implicated in 0.5% of adult cases of obesity.10
Until recently, the genetic etiology of obesity in the general population was elusive. However, large-scale genome-wide association studies, which examine the variation of frequency of thousands of alleles with disease status in very large samples, have led to the discovery of FTO (fat mass and obesity–associated gene) as an obesity-susceptibility gene.11 These associations, which have been replicated across populations, indicate homozygotes for the risk allele weigh on average 3 to 4 kg more and have a 1.67-fold increased risk of obesity compared with persons without the risk allele. Although the functioning of this gene is not well understood, it is believed that FTO confers a risk of increased obesity risk through regulation of food intake, and possibly via mechanisms that influence stress responses. Other genetic variants, which might individually explain less than 4% of the phenotypic variation, include polymorphisms in genes involved in catecholamine function, mitochondrial respiration, and various pathways involved with insulin sensitivity.8
Craniofacial Morphology
Craniofacial morphology, which encompasses both bony and soft tissues, is believed to predispose to OSAH by reducing upper airway patency. Structural features that have been described in patients with OSAH using techniques such as cephalometry include reduction of the anterior-posterior dimension of the cranial base, a reduced nasion-sella-basion angle, reduction of the size of the posterior and superior airway spaces, inferior displacement of the hyoid, elongation of the soft palate, macroglossia, hypertrophy of adenoids and tonsils, increased vertical facial dimension with a disproportionate increase in the lower facial height, and mandibular retrognathia or micrognathia.12–14 (see Chapter 105). A brachycephalic head form, measured by anthropometry, is often found in association with reduced upper airway dimensions. This head form is associated with a small but significant increased risk of OSAH in whites, and it also identifies families at risk for both OSAH and sudden infant death.15 In African Americans, this head form is uncommon and does not appear to increase risk of OSAH.
In humans, the genetic basis for craniofacial features is supported by both twin and family studies.16 Over one third of the variability in the volume of soft tissue airway structures including the tongue and lateral pharyngeal walls can be explained by familial factors.17 There are also at least 50 syndromes in which congenital malformations of mandibular and maxillary structure occur, many of which also are associated with respiratory impairment and upper airway obstruction. These include Pierre-Robin syndrome and Treacher Collins syndrome.18,19 Studies of various syndromes and genetic defects suggest potential roles of genes belonging to the fibroblast growth factor (e.g., FGFR1, FGFR2, FGFR3), transforming growth factor beta (e.g., TGFBR1, TGFBR2), homeobox (e.g., MSX1, MSX2), and sonic hedgehog (e.g., PTCH, SHH) pathways. Other potentially relevant candidate genes are those that have been implicated in craniofacial development, including genes on the endothelin pathway (e.g., ECE1, EDN1, EDNRA),20–22 and TCOF1, the cause of Treacher Collins syndrome.23 Further understanding of homeobox genes and genes controlling growth factors might contribute to our clarifying the origins of craniofacial dysmorphisms found in OSAH.
Inherited abnormalities of craniofacial structure appear to explain at least some of the familial aggregation of OSAH. Relatives of OSAH probands have been shown to have decreased total pharyngeal volumes and glottic cross-sectional areas, retropositioned maxillas and mandibles, and longer soft palates compared with relatives of controls.24 Relatives of patients with OSAH have been shown by cephalometry to have a more retropositioned mandible and smaller posterior superior airway space compared to normative data.25 In the Cleveland Family Study, both hard tissue (e.g., head form, intermaxillary length) and soft tissue (e.g., soft palate length, tongue volume) factors predicted the AHI level in European Americans. In African Americans, soft tissue factors also predicted AHI levels, but hard tissue anatomic features appeared to be only weakly associated with OSAH.26 These data support the importance of structural features in increasing susceptibility to OSAH, but they also suggest that the anatomic underpinnings and the genes for upper airway anatomy might differ among ethnic groups.
Magnetic resonance imaging (MRI) (see Chapter 101) studies have shown that the lateral pharyngeal wall and tongue are larger in OSAH patients compared with matched controls.27 More than one third of the variability in the volume of soft tissue airway structures including the tongue and lateral pharyngeal walls was estimated to be explained by familial factors.17
Although MRI precisely describes anatomic characteristics, its cost limits its utility for large-scale genetic epidemiology studies. An alternative approach is use of acoustic reflectometry, which is a noninvasive technique for assessing airway cross-sectional area as a function of airway distance, and has identified associations of OSAH with mean and minimal airway cross-sectional areas.28–31 The utility of acoustic reflectometry for phenotyping airway risk factors is supported by a study of 568 participants in the Cleveland Family Study. In this sample, the heritability estimate for minimal cross sectional area in both European Americans and African Americans was .37 (P < .05), which increased to .55 once only the highest-quality curves were analyzed.32 These estimates were not appreciably changed after considering the influence of BMI, suggesting that between 30% and 55% of the familial similarities in airway dimensions may be explained by genetic factors apart from BMI. Future research needs to assess the ability of such techniques to identify subgroups of persons who inherit polymorphisms associated with genes on craniofacial as compared to other etiological pathways, and further investigation of how anatomic measurements performed awake and in the sitting position predict collapsibility during sleep.
Ventilatory Control Patterns
Mouse models have allowed genes to be identified that influence control of ventilation, including genes that determine respiratory timing, frequency, awake ventilation, chemosensitivity, and load responses. Clear strain differences have been observed for many of these phenotypes, with evidence of quantitative trait loci near plausible candidate genes. Knockout and transgenic mice also have helped identify the role of specific proteins and receptors in ventilatory chemoreception, neuromuscular transmission, and neural integration. Candidate genes identified from such studies include genes that sense hypoxia33–36; genes on the endothelin pathway,37,38 which also are important in craniofacial development; and genes that regulate neural crest migration,38–40 including PHOX2B, mutations of which cause congenital central hypoventilation.41,42
The magnitude of ventilatory chemoresponsiveness appears to be subject to major genetic control; heritability estimates for chemoresponsiveness to oxygen saturation levels range from approximately 30% to 75%.43 Ventilatory responses are more strongly correlated between monozygotic than dizygotic twins.44–47 Population differences in ventilatory patterns and hypoxic sensitivity have been identified for populations that have adapted to living at high altitude.48,49 Abnormalities in hypoxic or hypercapnic ventilatory responsiveness have been described in the first-degree relatives of probands with unexplained respiratory failure,50 chronic obstructive pulmonary disease,51,52 and asthma.53 Unfortunately, further identification of the role of ventilatory control as an OSAH intermediate phenotype has been impeded by the overall complexity in measuring relevant ventilatory control abnormalities in humans.
A potential role for inherited impairments of ventilatory control in influencing susceptibility to OSAH has been suggested by several studies of carefully characterized families. These studies have demonstrated blunted hypoxic responses and impairment in load compensation in the families of OSAH patients compared with controls. El Bayadi and colleagues reported the results of anatomic and physiologic studies performed in 10 subjects from three generations of a family with an affected proband with OSAH.54 OSAH was documented in 9 of the 10 family members, all of whom had a body mass index less than 30. Blunted responses to ventilatory challenge tests to progressive hypoxia were demonstrated in all five subjects who underwent ventilatory challenge testing. Cephalometry also showed variable degrees of upper airway anatomic compromise. These findings supported a family basis for OSAH, with evidence that inherited abnormalities in anatomic and physiologic risk factors both contributed to the disease severity. Significantly lower ventilatory responses to hypoxic challenge testing were demonstrated in a study that compared ventilatory control responses in 31 subjects from 12 families with two or more members with OSAH, compared to responses in nine age- and sex-matched controls.55 The selection of subjects from families showing familial aggregation for OSAH might have improved the ability to detect potentially inherited abnormalities in an intermediate phenotype.
In another study, differences in responses to inspiratory resistive loading during sleep were examined in 10 apparently healthy (without OSAH) adult offspring of OSAH probands and in 14 control offspring of healthy parents.56 Both groups had similar load responses during wakefulness, but during sleep with inspiratory loading, the offspring of OSAH probands breathed at a lower tidal volume than controls, with development of hypopneas at lower levels of resistance, and were less likely to show EEG arousal with hypopneas than the controls. Similarly, nasal occlusion during sleep was demonstrated to precipitate more hypopneas in the healthy relatives of OSAH probands than controls.57 However, these studies could not differentiate the extent to which differences in ventilatory patterns between the groups was due to differences in collapsibility versus ventilatory drive.
Javaheri and colleagues tested for differences in chemoresponsiveness to progressive hypercapnia and to hypoxia in the healthy relatives of hypercapnic OSAH patients compared to eucapnic OSAH patients.58 Although the chemoresponses among relatives were correlated, the relatives of patients with and without hypercapnia did not differ. Thus, this study did not provide evidence for genetically determined differences in chemoresponsiveness influencing the propensity of hypercapnia in OSAH.
The potential impact of deficits in ventilatory control on OSAH susceptibility is likely magnified in persons with anatomically comprised upper airways. With sleep onset, the central inspiratory drive to upper airway motor neurons, a major determinant of airway patency, is reduced or fluctuates.59,60 Any given reduction in central inspiratory drive results in greater increases in upper airway resistance in persons with anatomically compromised airways than in others.61 Conversely, persons with greater degrees of upper airway resistance (due to craniofacial or obesity risk factors) can require a high level of compensatory ventilatory drive to overcome sleep-associated airway collapse, and thus they may be especially vulnerable to the influence of genetically determined ventilatory control deficits. These observations underscore the potential importance of considering the interaction of genetic risk factors that influence more than one etiologic pathway.
Control of Sleep and Circadian Rhythm
Abnormalities in orexin genes, or genes coding for their receptors, could be relevant to studies of OSAH because of the potential impact of these neuropeptides on arousal and muscle tone, both of which influence the behavior of respiratory systems, because of the close proximity of these neurons to central respiratory control centers, with potential interactions between arousal and respiratory centers. Orexins have also been shown to play a role in energy homeostasis and the regulation of feeding and projections to areas in the ascending cortical activating system, are involved with regulation of both appetite and sleep–wake states,62,63 as well as ventilatory control.64 Although this pathway has not been well studied in OSAH, orexin A levels are reported to be reduced in OSAH.65 Thus, abnormalities in orexin genes may be relevant to OSAH because of their influence on arousal, muscle tone, ventilatory control, and weight control. Data from the Cleveland Family Study indicate that an area on chromosome 6 that houses the orexin-2 receptor is linked with the AHI (see later).
In addition to considering the impact of genetic abnormalities on processes that regulate sleep–wake state, it may be useful to consider how respiratory motor neuron control may be influenced by genetic processes that determine circadian clocks, which are known to drive important metabolic and behavioral rhythms. Studies of Drosophila and mouse models have identified a number of genes that influence periodicity and persistence of circadian rhythms,66–69 with evidence that similar mechanisms operate in humans.69,70 The relevance of these findings to OSAH is unclear. However, genetic variations in regulation of sleep–wake rhythm may be factors that influence the expression of OSAH (e.g., ability to compensate or show sleepiness in response to recurrent apneas and sleep disruption).
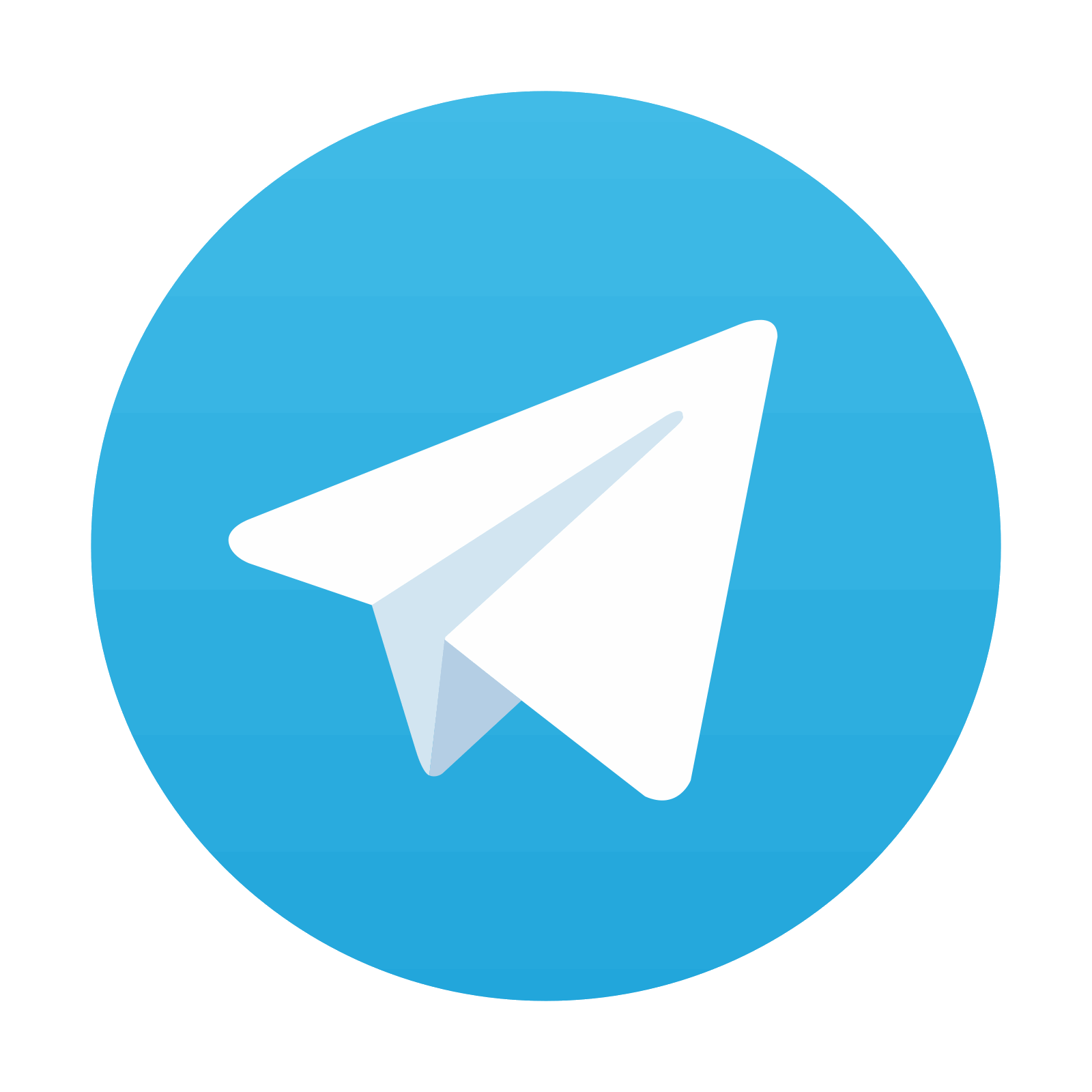
Stay updated, free articles. Join our Telegram channel
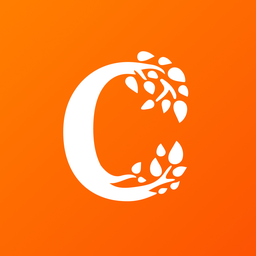
Full access? Get Clinical Tree
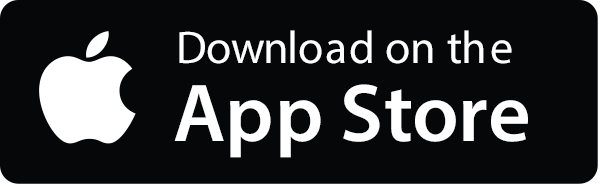
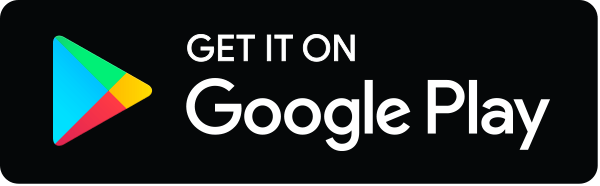