Higher Motor Control
Now we need to have a look at the levels of the motor system where we start to interpret and apply the detailed information delivered through the special senses. In ascending hierarchical order, these are the primary motor cortex, the cerebellum, the basal ganglia and prefrontal cortex.
The task of the motor system
Students often find the higher levels of the motor system difficult to understand: so do those who do research on them. As we have already seen (p. 189), there are peculiar experimental difficulties with investigating motor systems in conventional ways. At the same time the anatomy of the pathways is complex and open to many interpretations (compare it with that of the visual system, for instance). And finally, and perhaps most important of all, the complexity of the movements themselves is extraordinarily hard to grasp. Think what is involved in one of the most familiar sequences of actions of all, getting dressed in the morning. Faced in semi-darkness with a crumpled, partially inside-out, shirt, what astonishing feats of computation are needed to work out how to pick it up, manipulate into the right configuration, guide it over the correct bits of one’s anatomy, deal with buttons and buttonholes. This is not a peculiarly human ability, not particularly dependent on primate-sized cortex: you only have to see a bird elegantly swoop between the branches of a tree, killing its speed with exactly the right timing to come to rest on one particular twig, to realize that equally daunting motor tasks can be accomplished with remarkably little neural hardware.
At the end of Chapter 9, we introduced the concept of hierarchical organization, and pointed out its utter inevitability in any system – a business enterprise, a military unit, a computer program – that is intended to carry out effective action. The areas of the motor system – cortex, cerebellum and basal ganglia – that we are about to look at are complex, ambiguous and poorly understood. The only way to make sense of them is – as always – to think functionally, and to be clear in one’s mind about the problems that the brain faces in trying to generate meaningful movements. Thinking hierarchically turns out to be particularly enlightening, since the structures concerned happen to fall into a hierarchical order that has a natural, almost inevitable, relationship to different aspects of the movements themselves.
The three components of voluntary action
One way of thinking about movement that many students find helpful is first to consider a familiar, tangible, representative, action – and then to break it down into its components. A good example is reaching out to take a cake from a plate on a table. Starting at the end – always the best plan in neurophysiology – we have grasping. The force in the fingertips must be carefully regulated to grip the cake safely without crushing it, despite its soft plasticity. There must be immediate sensing of any slip, and immediate adjustments must slightly increase the pressure of grip. Its weight must be estimated so that appropriate lifting forces are applied: it may perhaps stick to the plate. But before all these mechanisms of manipulation come into play, we must have succeeded in reaching it, an operation demanding an entirely different set of computations and control mechanisms. During the reaching the position of the cake must be monitored, using visual information supplemented by knowledge of eye position relative to the head, and head position relative to
the trunk. Meanwhile, we need constant proprioceptive feedback from our limbs so that we can match the position of our hand to that of the cake. At the same time, we need to make slight postural adjustments to counteract the destabilizing effect of extending the arm. And preceding both reaching and grasping come the processes of decision and planning. Are we actually hungry? Do we want the pink cake or the yellow one? Do social conventions either permit or forbid taking the cake at all? What route will avoid knocking over that milk-jug?
the trunk. Meanwhile, we need constant proprioceptive feedback from our limbs so that we can match the position of our hand to that of the cake. At the same time, we need to make slight postural adjustments to counteract the destabilizing effect of extending the arm. And preceding both reaching and grasping come the processes of decision and planning. Are we actually hungry? Do we want the pink cake or the yellow one? Do social conventions either permit or forbid taking the cake at all? What route will avoid knocking over that milk-jug?
![]() |
By a happy coincidence, these logically distinct stages of planning, reaching and grasping – which amount to what, where and how – happen to correspond with precisely those three main areas that we shall be looking at in this chapter. Grasping is the peculiar province of the primary motor cortex, which receives precisely the kind of information need to manipulate objects, and has the right descending connections to be able to control force and velocity with precision and speed. It is here that desired positions of the limbs and so forth may be translated into detailed instructions about the forces required from moment to moment in order to achieve these results. Patients with damage to this region, or indeed impairment of the sensory systems relaying the requisite information from the skin, can plan and reach perfectly well, but are clumsy when it comes to the last stage of actually grasping and lifting, particularly when the thing being picked up is unfamiliar. For patients with damage to the cerebellum, on the other hand, the problem is mostly in the reaching: distances are misjudged, with overshoot and oscillation, and the trajectory may be broken down into a sequence of more tractable sub-components. The hand has to be guided on to the target with conscious effort. Finally, with hypokinetic disorders of the basal ganglia such as Parkinson’s disease, movements may be well executed if they are executed at all, but the patient is conscious of the difficulty of getting them started, or sometimes of stopping them once they are under way. And frontal precortex damage may encourage cakesnatching, socially inappropriate but deftly executed – an error of decision rather than of planning, reaching or grasping.
![]() |
Primary motor cortex

two or three subdivisions, with distinct functions, but the terminology is still confused. There is also a quite separate supplementary motor area (called M2, the first being MI; it is also known as the secondary motor area, SMA) which, like the corresponding sensory area S2, is more bilaterally organized than the strictly contralateral MI.

![]() |
![]() |
Two thalamic nuclei are paired in this way with the cortical motor areas: they are the ventrolateral nucleus, which projects mostly to area 4 and receives fibres back from both area 4 and the somatosensory cortex; and the ventroanterior nucleus, which projects to area 6 and receives returning connections from both area 4 and area 6. The ascending input to the motor nuclei of the thalamus is not sensory, but comes partly from the basal ganglia and cerebellum and partly from the reticular formation; these regions also project to the more diffuse centromedial thalamic nuclei, which communicate more widely with both somatosensory and motor cortex, and other areas as well. The output from the sensorimotor regions is diverse: some of course makes up the corticospinal tract (CST), some can reach the cord indirectly via the red nucleus, colliculus, and nucleus gigantocellularis of the reticular formation, but most project to the basal ganglia and – via relays in the pons and inferior olive – to the cerebellum. These indirect routes are sometimes lumped together under the heading of extrapyramidal pathways, those outputs that do not simply go straight down into the spinal cord. 

![]() |
Cortical influence on the spinal cord
Area 4 is differentiated from all other areas of the cortex by having a number of particularly big cells in layer V, the giant Betz cells. Their size suggests that they might be the origin of the corticospinal tract, and for a long time the notion was current that the essence of the voluntary motor system was something like what is shown below (A), with ‘volition’ somehow triggering off the Betz cells of Area 4, which in turn synapsed directly with spinal motor neurons: the former were called the ‘upper motor neurons’ and the latter the ‘lower motor neurons’, implying that the effects of stimulating the motor cortex were entirely due to stimulation of the cortical tract.
![]() |
This is an unhelpful and misleading picture in a number of ways. In the first place, it is clear that the Betz cells are not the sole origin of the corticospinal tract: for one thing, the latter contains about a million fibres, whereas there are only some 30 000 Betz cells. It is not even true that the tract comes only from the motor cortex: in primates about 30 per cent or less comes from area 4, another 30 per cent from area 6, and the rest from more posterior areas, somatosensory and parietal (B). Nor is it true that the effects of cortical stimulation are even mainly due to activation of corticospinal fibres. If in the monkey one traces the motor map by electrical stimulation both before and then after completely severing the pyramidal tract, the distribution of responses is found to be essentially unchanged (though they may tend to be a little slower and require a larger current to be evoked). And finally,
as we have already seen, even in the primates only some of the corticospinal fibres end directly on motor neurons, while in other species none do: cortical control is probably rather of spinal circuits than of individual muscle units. The CST is not a particularly rapid conduction route: some 5 per cent are unmyelinated in Man, and of the myelinated fibres, 90 per cent of the fibres are quite small, with diameters around 1-4 µm.
as we have already seen, even in the primates only some of the corticospinal fibres end directly on motor neurons, while in other species none do: cortical control is probably rather of spinal circuits than of individual muscle units. The CST is not a particularly rapid conduction route: some 5 per cent are unmyelinated in Man, and of the myelinated fibres, 90 per cent of the fibres are quite small, with diameters around 1-4 µm.
Lesions of the corticospinal tract give similar, but not identical, effects to lesions of the cortex itself: no gross defect, but generally some hypotonia and weakness (paresis), and more specifically loss of skilled movements, particularly of extremities such as the fingers, as in picking up a small object; lesions of area 4 are in general rather more severe, resulting at first in a wholly flaccid paralysis, and typically in greater functional loss than pyramidal section by itself, as the figure implies. When recovery has reached completion there is typically a degree of residual clumsiness, reminiscent of what is seen when feedback from the periphery is impaired (see Chapter 10, p. 212). There are certain difficulties of interpretation, however, both by reason of the marked species variation that is seen – a cat can still apparently run about after complete resection of its pyramids – and also because gradual (though variable) recovery is a feature of lesions of both cortex and pyramidal tract. In time, the immediate flaccidity resulting from a lesion in area 4 usually develops into a spasticity, presumably through some kind of release phenomenon. Lesions that are large enough to encroach on area 6 as well as 4 also tend to produce spasticity, which might be interpreted as suggesting that area 6 has some kind of tonic inhibitory action, a notion reinforced by observations of the effects of stimulating area 6 during voluntary or evoked movements. At all events, the classical clinical description is that an ‘upper motor lesion’ gives a spastic paralysis, without muscle wasting, while a ‘lower motor lesion’ produces a flaccid paralysis. In summary, the CST is only partly from area 4; area 4 projects only partly to the CST.
Studying the relationship between motor neurons and pyramidal cells serves to exemplify how three techniques pivotal to studying neurophysiology complement each other:
Stimulation: extracellular stimulation of cortical neurons reveals systematic representation in the cortex, analogous to that found in somatosensory or visual cortex, with groups of neurons corresponding to synergistic muscles found co-localized in columns. Stimulation of such a column generates coordinated activation of synergists. Furthermore, stimulation with intracellular electrodes indicates clearly that each one of the pyramidal tract neurons excites only one muscle at a time, either directly (most obviously in Man), or via the specific facilitation (or sometimes inhibition) of one particular stretch reflex.
Reverse mapping: peering back up at the cortex from an individual motor neuron in the cord, we can identify the population of pyramidal cells making a monosynaptic connection with an individual motor neuron. In the monkey such a population can be mapped back to an area in the primary motor cortex of some 10 mm in diameter.
Recording: conversely, recordings from individual pyramidal cells during spontaneous directed movements in monkeys show that movements are encoded by populations of neurons. The final direction of a movement is the result of a kind of weighted average of the activity in an ensemble of cortical neurons, an arrangement which – for reasons which are, as it were, the mirror image of the reasons given in Chapter 4, for the desirability of overlap of fields in sensory systems – gives robustness and sensitivity to small changes; conceptually, the ratio of activity of a ‘move right’ and a ‘move left’ neuron could elicit movement in any vector between ‘right’ and ‘left’, rather than having a neuron for every conceivable direction.
Somatosensory input and manipulation
One of the most interesting findings comes from using the same microelectrode for recording as well as stimulation. What is found is that monkey pyramidal cells have very wide, multimodal receptive fields, from joint receptors and tendon organs as well as skin receptors, and with a small representation of spindle afferents as well. If one looks at the relationship between what any particular cell does when it is stimulated and what it actually responds to, it is striking that frequently the cutaneous receptive fields represent those areas of skin that are normally brought into contact with one another when that particular muscle contracts, and are found to be most active during precision grip rather than power grip. This strongly suggests that the cortex is the site of the kinds
of sensorimotor correlation required whenever we are grasping, touching or manipulating our environment.
of sensorimotor correlation required whenever we are grasping, touching or manipulating our environment.
![]() |
That this is perhaps the most important single function of the primary motor cortex is suggested also by the relative sizes of different parts of the body in the motor map. In Man, only two areas of the body are used to any large extent for tasks of this kind that require accurate feedback from the skin, namely the hands and the mouth; and both of these together form by far the greatest proportion of the motor map. As shown in the highly schematic figure below, in the monkey, which makes more use of its feet for handling objects, they have almost as large a representation as the hands, and relatively much larger than in Man. In most four-footed animals it is only the mouth and lips that are used for grasping and exploration, and their representation is correspondingly enlarged; virtually the whole of the pig’s motor cortex is said to be devoted to its snout! It is also perhaps significant that lesions in area 6 – which we saw to be predominantly inhibitory in effect – often produce uninhibited grasping of the kind frequently observed in very young children: any object touched against the palm results in immediate forceful seizure, with an unwillingness to let go. Destruction of the motor cortex also abolishes the tactile placing reaction described in Chapter 11.
![]() |
One further function of primary motor cortex, of a related kind, is probably the generation of the ‘expected force’ commands that were described in Chapter 10. We saw there that these commands need to be adjusted to match the load that is to be encountered, and that one source of information about load that seems to be used to do this comes from pressure receptors in the skin; tendon organs, which as we have seen also project to pyramidal cells, are another likely source of information about load (see p. 207). Could it be that the pyramidal tract fibres are the route by which force commands are sent to the spinal cord? They certainly have the right kinds of information at their disposal to carry out such a function, and also appear to give the expected effects of facilitating stretch reflexes when stimulated. And if one records from the pyramidal tract of a conscious freely moving animal, and observes the circumstances under which pyramidal fibres actually fire during voluntary movements, one finds that whereas the correlation between pyramidal activity and muscle length or velocity is poor, there is a clear correlation between frequency of firing and the force that is being produced at any moment to generate the movement, suggesting very strongly that this tract does indeed provide force commands.
Finally, we have seen that the effect of lesions to motor cortex or pyramidal tract is not just a loss of the kinds of skilled movements that require close coordination between skin sensation and movement, but also a general weakness of the muscles, with a correspondingly increased sense of effort on the part of the patient. Sense of effort seems to be found in situations where the gain of stretch reflexes is insufficient, for example in the experiments described on p. 211 in which the pressure receptors in the subject’s thumb were blocked by local anaesthesia, and may well be associated with voluntary excitation of motor cortex neurons. Partial paralysis tends to result in an apparent increase in the heaviness of a weight being lifted.
If it is true that the main function of the motor cortex is a relatively simple coordination between skin and other spinal afferents and the gain of stretch reflexes, one might well wonder why this function has to be carried out in the cortex and not in the cord itself, where the inputs and outputs actually are. Why bother to go all the way up to the top of the head? There are probably two reasons why the spinal cord is essentially unsuited to the task. The first is that most spinal reflexes seem to be organized in a segmental manner. But the feedback from skin receptors resulting from contraction of a particular muscle will not always return to the cord via the dorsal roots of the same segment; in the cortex, however, information from different segments is brought into much closer approximation, along with information from other sources such as the visual and vestibular system. The large amount of convergence and divergence – in the monkey, each pyramidal cell receives some 60 000 synapses – means that connections from different inputs can presumably be allocated more easily to their appropriate muscles, rather as subscribers’ lines converge on a telephone exchange. Thus although the primary motor cortex is near the bottom of the motor hierarchy, physically it has to be near the top, a cortical area. It is instructive to compare its role in controlling the hand with what the reticular formation does in
controlling the eye: because eye muscles have a constant load, they do not need the same range of feedback from mechanoreceptors, and the ‘How’ part of their control does not have to be cortical.
controlling the eye: because eye muscles have a constant load, they do not need the same range of feedback from mechanoreceptors, and the ‘How’ part of their control does not have to be cortical.
![]() |
The second point is that it is difficult to see how appropriate connections could ever be set up in the cord in the first place: how can an incoming sensory fibre from the skin know which of the hundreds of thousands of ventral horn cells are the ones to which they are supposed to make contact? It seems much more likely that such connections are established through experience, by frequent association of stimulation of a particular afferent with contraction of a particular muscle. This implies a richness and flexibility of connections, an ability to form functional contacts as the result of experience, which the cortex seems specifically adapted to perform, through the same types of memory-like mechanisms suggested in Chapter 7, in the case of the visual cortex. And just as depriving sensory input can cause large and rapid alterations in the relative areas of somatosensory cortex devoted to different regions of the body, similar changes can be demonstrated in primary cortex by stimulation or by reduction of use.
Finally, it should not be forgotten that if it is convenient for the control of movement to have cutaneous information readily available in areas 1, 2 and 3 right next door to the motor cortex, it is equally important in analysing cutaneous sensations to have information immediately on hand about what movements are being executed. It was emphasized in Chapter 4 that such common sensations as softness, resilience, roughness, stickiness, and the like rely on knowledge of what forces are being applied to the surface in question by the motor system, knowledge that is easily supplied by pyramidal tract neurons, through the rich interconnections between the two cortical areas. In human patients, electrical stimulation of the motor cortex produces sensory effects not very different from those resulting from stimulation of the somatosensory cortex: numbness, tingling, and a sense of movement which may or may not be accompanied by actual movement; if it is, the subject feels that he has been ‘made’ to carry out the action by the experimenter rather than ‘wanting’ to make it.
Secondary motor cortex
The functions of the two secondary areas, SMA and PMA, are much less well understood. Lesions in the supplementary motor area seem to cause a specific detriment in internally generated movements made from purely motor memory, though the animal can still learn the same movements in response to external signals. This is analogous, as we shall see, to what is observed in certain kinds of disorder of the basal ganglia (from which SMA receives input), and suggests a possible functional linkage between the two. Electrical stimulation of SMA can produce movement, and recording in conscious monkeys has shown a specific activation of SMA in complex tasks, especially those involving carrying out a sequence of actions from memory. Stimulation in parts of PMA also produces movement, and lesions in certain parts of it suggest a clear distinction between SMA and PMA, that the former is on the whole more concerned with internally triggered movements and is related to the basal ganglia, the latter with movements guided by external stimuli, and related to the cerebellum (from which it receives a great deal of input). However, this is a very active area of research, complicated by difficulties of identifying and classifying the functional boundaries of the areas themselves, and by uncertainties in relating observations in monkeys to what happens in the human. 

Cerebellum

![]() |
The cerebellum seems to have grown out of the brainstem as an adjunct to the vestibular system, in effect to provide it with some intelligence; but this oldest part of it, the archicerebellum or vestibular cerebellum, is now dwarfed by two newer areas: the palaeocerebellum associated with the spinal cord, and hence also sometimes called the spinocerebellum, and the large and central neocerebellum or corticocerebellum, whose development has been in step with that of the cerebral cortex from
which most of its input is derived. These areas are best appreciated when the cerebellum is ‘unrolled’, as below. The cortex of the cerebellum has a very regular and beautiful neuronal structure quite unlike the chaos seen practically everywhere else in the central nervous system, which has led to it being called ‘the neuronal crystal’: so machine-like that it is impossible not to feel one ought to be able to work out what it is doing with just a little thought. Consequently it has provoked more speculation than anywhere else in the brain – particularly from mathematicians and computer scientists – about how its circuits might work. To put it another way, if we can’t even work out what the cerebellum is doing, what hope is there anywhere else?
which most of its input is derived. These areas are best appreciated when the cerebellum is ‘unrolled’, as below. The cortex of the cerebellum has a very regular and beautiful neuronal structure quite unlike the chaos seen practically everywhere else in the central nervous system, which has led to it being called ‘the neuronal crystal’: so machine-like that it is impossible not to feel one ought to be able to work out what it is doing with just a little thought. Consequently it has provoked more speculation than anywhere else in the brain – particularly from mathematicians and computer scientists – about how its circuits might work. To put it another way, if we can’t even work out what the cerebellum is doing, what hope is there anywhere else?

![]() |
![]() |
Cerebellar neurons and their connections
The most conspicuous type of cell in the cerebellar cortex is the Purkinje cell, which with silver staining displays a huge dendritic tree that is confined to a plane perpendicular to the surface and roughly anteroposterior. The cells are lined up in soldierly rows over the whole of the cortex, with their dendritic trees thus stacked like a pack of playing cards. There are about 15 million of them, and each has a dendritic surface area equivalent to two average-sized front doors.
Deep nuclei
The Purkinje cells form the sole output of the cerebellar cortex, for their fibres run downwards to the deep nuclei that lie in the core of the whole structure. In primates there are four of these nuclei on each side (fastigial, emboliform, globose and dentate); in other species the second and third of these are fused into one, called the interpositus. In one region – the flocculo-nodular lobe – the Purkinje output is not to a ‘deep nucleus’ but to part of the ipsilateral vestibular nuclei.
![]() |
The projection from the cerebellar cortex on to these nuclei is a systematic one: medial areas (blue, above) project to the fastigial region, lateral ones (green) to the dentate, and the intervening region to the interpositus. A curious feature of the Purkinje cell is that although it is the only output from the cortex, it is entirely inhibitory (the transmitter is γ-aminobutyric acid (GABA)) on the deep nuclei. Finally, the deep nuclei themselves project to various other motor structures: the fastigial primarily to the vestibular nuclei, interpositus to the red nucleus, and dentate to the thalamus (mainly ventrolateral) and hence to the cerebral cortex; all probably also send fibres to the reticular formation of the brainstem. Thus there are several routes by which the cerebellum can influence the spinal cord.
Climbing fibres
Afferent information reaches the Purkinje cells by two quite distinct pathways. In the first place, each Purkinje cell receives a unique single fibre that climbs up its efferent axon and then branches to clamber all over its dendrites like ivy on a tree, forming synaptic contacts that are believed to release aspartate and are very strongly excitatory. These climbing fibres come from the
inferior olive in the brainstem, which receives its input in turn mostly from the cerebral cortex, but also from the spinal cord and to some extent from the special senses (as, for example, from the visual system, in the case of the vestibulocerebellum); as well as these contacts with Purkinje cells, they also excite the deep nuclei to which the Purkinje cells project. Such specificity of synaptic contact with a single cell is rather a rarity in the central nervous system, where wide divergence and convergence seems to be the general rule, and experimentally it is found that a single shock to a climbing fibre never fails to fire the associated Purkinje cell, sometimes repetitively. However, it is important to realize that Purkinje cells are normally extremely active at all times, including at rest, whereas climbing fibres seem to fire only infrequently (perhaps once a second on average, if ‘average’ means anything in this context). On the other hand, the extensive intimacy of contact between climbing fibre and Purkinje cell, and the latter’s unusual electrophysiology (with voltage-gated calcium channels in its dendrites) means that a single climbing fibre impulse can have a highly significant effect on the Purkinje cell, triggering a ‘complex spike’ (actually a burst of spikes followed by a period of quiescence) that is capable of signalling to the whole dendritic tree, as is required if remote synapses from parallel fibres are to be capable of Hebbian plasticity (see below, p. 259).
inferior olive in the brainstem, which receives its input in turn mostly from the cerebral cortex, but also from the spinal cord and to some extent from the special senses (as, for example, from the visual system, in the case of the vestibulocerebellum); as well as these contacts with Purkinje cells, they also excite the deep nuclei to which the Purkinje cells project. Such specificity of synaptic contact with a single cell is rather a rarity in the central nervous system, where wide divergence and convergence seems to be the general rule, and experimentally it is found that a single shock to a climbing fibre never fails to fire the associated Purkinje cell, sometimes repetitively. However, it is important to realize that Purkinje cells are normally extremely active at all times, including at rest, whereas climbing fibres seem to fire only infrequently (perhaps once a second on average, if ‘average’ means anything in this context). On the other hand, the extensive intimacy of contact between climbing fibre and Purkinje cell, and the latter’s unusual electrophysiology (with voltage-gated calcium channels in its dendrites) means that a single climbing fibre impulse can have a highly significant effect on the Purkinje cell, triggering a ‘complex spike’ (actually a burst of spikes followed by a period of quiescence) that is capable of signalling to the whole dendritic tree, as is required if remote synapses from parallel fibres are to be capable of Hebbian plasticity (see below, p. 259).
![]() |
Mossy fibres
The other type of afferent system is utterly different. Here, the incoming fibres enter the lower layers of the cerebellar cortex and branch to form large terminal structures (giving them their name of mossy fibres) that synapse in glomeruli with a number of dendrites from the extraordinarily numerous granule cells in the cortex (there are some 1010 of them). These in turn send ascending axons to the surface, where they bifurcate and send two thin axons (called parallel fibres) in opposite directions, perpendicularly piercing the planes of the stacked Purkinje cells, to whose dendritic trees they form side-connections, like telephone wires on a telephone pole. In this way, and in complete contrast to the highly specific climbing fibres, each parallel fibre can contact a large number of Purkinje cells; conversely, each Purkinje cell receives nearly half a million contacts from parallel fibres. Mossy fibres carry information from a very wide range of sources: directly, from vestibular and spinal afferents, notably from muscle spindles, and visual, auditory and spinal afferents also indirectly through the precerebellar nuclei in the reticular formation (lateral reticular nucleus, the nucleus reticularis tegmentum pontis and the paramedian reticular nucleus), and finally from the cortex via relays in the pons. The receptive fields of Purkinje cells are very large indeed – sometimes extending over a whole limb – and are remarkably multimodal. This arrangement of parallel fibres piercing perpendicular sheets of flat Purkinje cells is an efficient way of providing the largest possible number of output channels with access to the largest number of input sources, within the smallest possible space.
It is quite instructive to compare this general arrangement with the other kind of cortex that is already familiar from Chapter 4, cerebral cortex. In both cases there are large output cells (here in black), with a great deal of associative input (red) forming modifiable connections with them. But in cerebral cortex, the input projects to a relatively circumscribed region, the column, whereas the cerebellum seems designed for a given mossy input to reach the maximum possible number of Purkinje cells. Another, very important, difference is that in the cerebellum there is a second and very specific kind of input, the climbing fibre, which is not associative but forces the Purkinje cell to fire; as far as we know there is no exact equivalent to this in cerebral cortex, though one could draw a broad analogy with the specific thalamic afferents. Just as the job of the cerebellum could be said to associate general patterns of activity in mossy fibres with specific and real errors signalled by the climbing fibres, so as to be able to predict them, so in cerebral cortex one could regard one of the functions
of a column as allowing general associative input from other columns, in order to learn to predict the activity in thalamic afferents that represents real events in the outside world.
of a column as allowing general associative input from other columns, in order to learn to predict the activity in thalamic afferents that represents real events in the outside world.
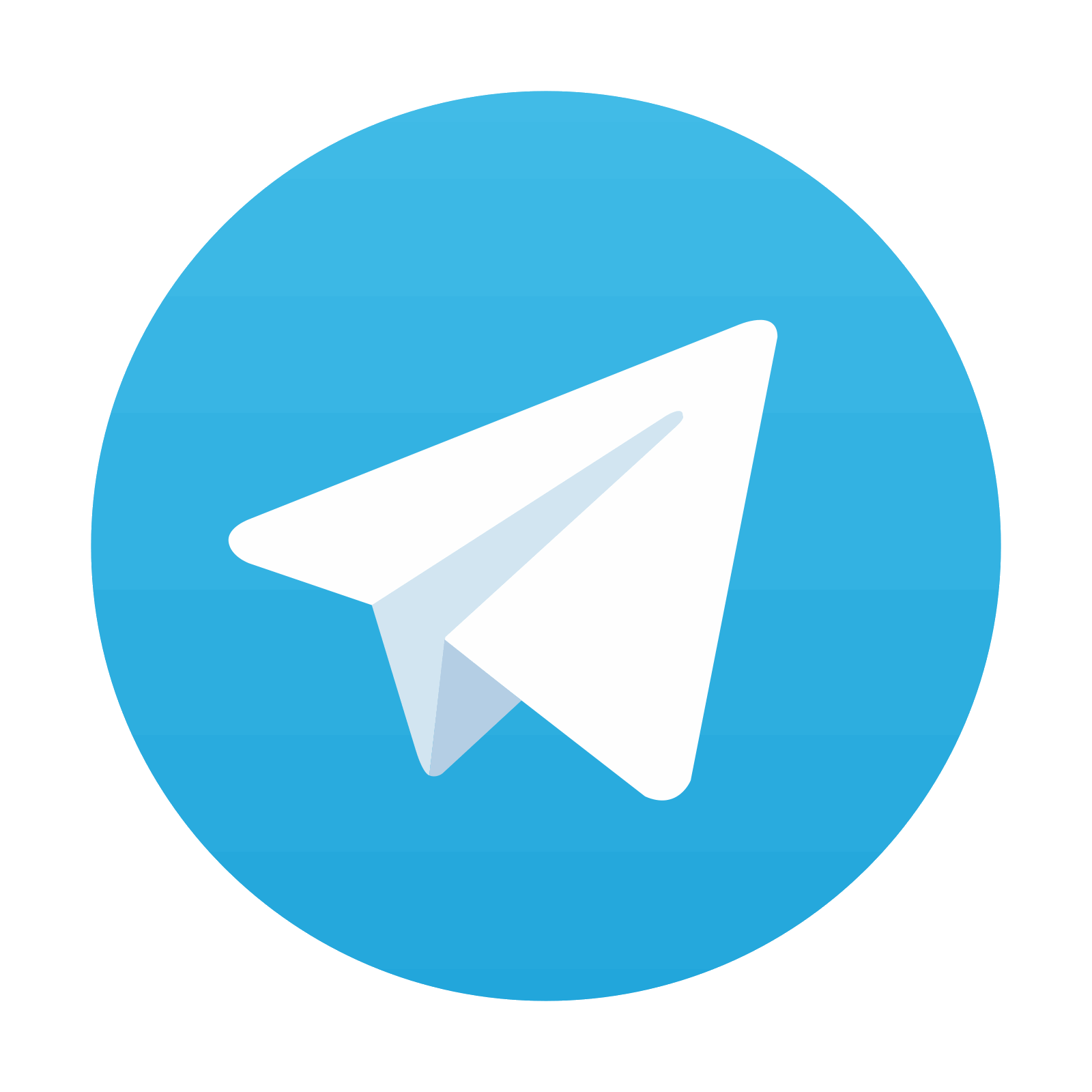
Stay updated, free articles. Join our Telegram channel
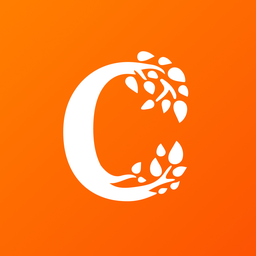
Full access? Get Clinical Tree
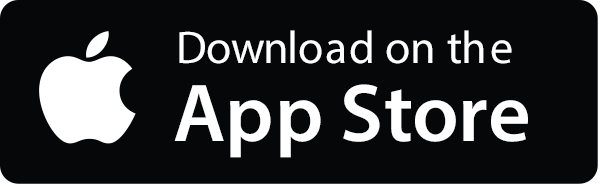
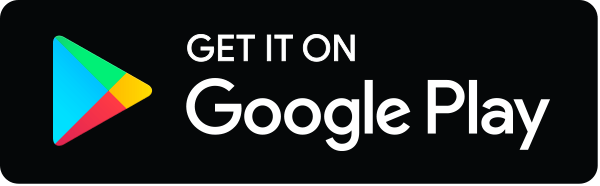
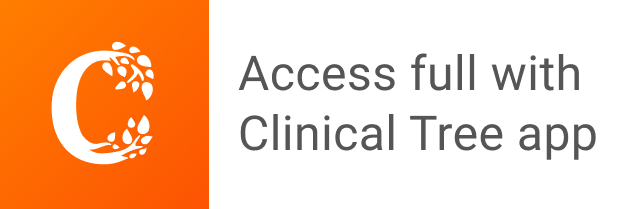