Hippocampal Sclerosis and Dual Pathology
Evan J. Fertig
Susan S. Spencer
Over the past several decades, technical advances have revolutionized the surgical treatment of medically refractory epilepsy, allowing precise localization and resection with higher rates of success and less morbidity. As a result, epilepsy surgery is increasing worldwide; however, estimates of the number of appropriate candidates far exceed actual referrals to epilepsy centers (1).
Mesial temporal lobe epilepsy (MTLE) associated with hippocampal sclerosis (HS) has served as a model for this treatment. The most common localization-related epilepsy, MTLE has well-known clinical, electrographic, and radiographic characteristics, is usually medically refractory, and is amenable to surgical correction (2,3). In the past 15 years, major epilepsy centers have published at least 25 studies, including the first randomized, controlled trial of surgical therapy (4,5), in which approximately two thirds of patients achieved control of disabling seizures, with low rates of morbidity and mortality. In properly selected patients with MTLE, therefore, surgery is superior to pharmacotherapy. Long-term outcomes for seizure control, neuropsychologic function, and quality of life, however, remain poorly characterized (6).
This chapter describes the current practice of surgical management for MTLE, beginning with its pathologic definition and including hypotheses of its significance and etiology, medical treatment and response, diagnostic evaluation, selection of candidates, surgical approaches and outcomes, and approach to bitemporal epilepsy. Modern imaging techniques have identified extrahippocampal lesions in association with HS, and surgery for this so-called dual pathology is also reviewed.
HIPPOCAMPAL SCLEROSIS
Pathologic Findings
Hippocampal sclerosis (HS), also known as mesial temporal sclerosis or Ammon horn sclerosis, is the most common pathologic finding in MTLE. Neoplastic, vascular, developmental, or traumatic abnormalities are found less frequently (2). Neuronal loss, gliosis, and reorganization classically define HS (7,8). Neuronal loss and gliosis primarily involve hippocampal sectors CA1, CA3, and CA4, with relative sparing of CA2 (2). Cell loss can extend into adjacent entorhinal cortex and amygdala (9). MTL neoplasms can also cause neuronal loss in the hippocampus, but usually less extensively than in classic HS (10).
A high proportion of astrocytes with a high density of sodium channels account for the gliosis. Although found in regions of cell loss, these astrocytes are not significantly correlated with neuronal loss. They, too, are abnormal and can generate neuron-like action potentials (8,11).
Resected hippocampi reveal neuronal reorganization in and around the “sclerotic” hippocampus. Selective loss of somatostatin and neuropeptide Y interneurons is seen, with sprouting of neuropeptide Y interneurons and dynorphin-staining granule cells into the inner third of the dentate molecular layer (8,12,13). Mossy fiber reorganization has also been found in human hippocampi removed for uncontrolled seizures (14).
Overall, this pattern is unique to MTLE with HS. The role of any of these pathologic changes in seizure generation, however, remains undefined.
Etiology
The high correlation of pathologic HS with ipsilateral epileptiform features on electroencephalography (EEG), together with excellent outcome after temporal resection, suggests that HS might cause, rather than only coexist with, MTLE. Its genesis is still unexplained.
Patients with HS often give a history of an early risk factor, such as a febrile seizure, trauma, or infection before age 4. Febrile seizures have long been proposed as the cause of HS, because retrospective studies have consistently linked them, especially if complex or prolonged febrile seizures (15, 16, 17, 18), with MTLE (19, 20, 21). Prospective studies of children with even prolonged febrile seizures have not, however, shown subsequent TLE (22,23). Nevertheless, the earlier the onset of seizures, the longer the seizure remission before emergence of intractable epilepsy (20).
Other evidence for HS as an acquired lesion comes from animal studies of sustained limbic seizures by intermittent stimulation of limbic pathways. Selective neuronal loss in CA1 and CA3 as well as gliosis and neuronal reorganization was observed; however, no spontaneous seizures occurred. Case studies in humans have found the development of HS after acquired lesions (24). However, many patients with chronic, uncontrolled epilepsy, including frequent generalized seizures, do not show features of HS (7,25), and patients who undergo electroconvulsive therapy are not at increased risk of epilepsy (26).
Another theory regards HS as the result of a developmental lesion, with febrile seizures the consequence of an underlying abnormality. Developmental migrational abnormalities are associated with HS (dual pathology) in 15% to 25% of cases; conversely, 10% to 15% of patients with HS also have cortical developmental abnormalities (2,27, 28, 29). HS can coexist with tumors, but usually only when they approximate the hippocampus; even then hippocampal cell loss is milder than with developmental lesions, suggesting a different mechanism (such as damage from local electrical propagation) (30). Overall, the severity of hippocampal cell loss is not proportional to the number of seizures or the duration of epilepsy. HS may thus be a preexisting lesion, rather than a sequela of damage from cumulative seizures (29, 30, 31).
Further evidence for a developmental cause of HS comes from three reports of children with MTLE who underwent surgical resection. Magnetic resonance imaging (MRI) demonstrated hippocampal atrophy, a known correlate of hippocampal cell loss, at the time of the first seizure before age 2 years. Increased T2 signal has not been seen before age 4 (27) and may reflect a secondary process, with hippocampal atrophy preexisting. Children over age 12 had pathologic hippocampal findings identical to those in adults. Children under 12 had cell loss confined to CA1 and the hilus, and no reorganization or sprouting. Sprouting age at surgery, and duration of epilepsy, but not degree of cell loss, were significantly correlated. The implication is that the 50% cell loss in CA1 and the hilus, together with gliosis, is an essential underlying element of HS at all ages. Sprouting and neuronal reorganization may be secondary and progressive, related to the duration of epilepsy. Increased T2 signal on MRI may be a correlate of the laterappearing neuronal sprouting or reorganization and is not necessary for development of MTLE. Indeed, hippocampal atrophy without T2 signal change is known to correlate with the typical clinical syndrome, electrographic characteristics, the classic pathologic features of HS, and a good surgical outcome.
It may not be possible to generalize the mechanism of HS in children and in patients of all ages. In one study (32), the incidence of dual pathology in children was 80%, far higher than in adults. This may explain the short delay between the initial insult and spontaneous unprovoked seizures in these patients. In later-onset MTLE, which has a longer latent period before spontaneous unprovoked seizures, epileptogenesis may occur more slowly by a different mechanism.
More evidence for a developmental model comes from families with MTLE and febrile seizures. Hippocampal volumes were measured in 23 members of two families, each including one member with MTLE, several members with only febrile seizures, and other individuals without febrile seizures or epilepsy (33). Both left hippocampal atrophy and T2 signal change were demonstrated in the person with MTLE. Left hippocampal atrophy but a normal signal was seen in all individuals with febrile seizures (but not MTLE) and in many of the seizure free family members. Unusual hippocampal architecture was consistent in each family but differed between the two families. These observations support the view of hippocampal atrophy as a developmental abnormality that facilitates febrile seizures and is sometimes associated with MTLE, perhaps related to other factors.
MEDICAL TREATMENT
Initial therapy for MTLE associated with HS is medical. Of all etiologies for partial epilepsy, partial epilepsy caused by HS has the worst prognosis for medical control, with only 10% to 42% of patients becoming seizure free (34,35). Moreover, an initial positive response does not necessarily predict a good long-term outcome. One-fourth of patients with medically refractory localization-related epilepsy have a significant period of seizure control and even remission before intractability is evident (20).
Greater success with newer antiepileptic drugs as monotherapy or in combination has not been documented (3,36).
A few patients may become seizure free after extended drug trials, but when to switch to a surgical approach remains unclear. Although medically refractory epilepsy has no standard definition, there is some evidence that complete control is highly unlikely after two antiepileptic drugs have failed (37). Surgical therapy is safe and efficacious; continued medical therapy poses risks of recurrent seizures, lost productive years, and higher mortality. Clearly an argument exists for early surgical therapy. A multicenter prospective study is examining this issue (38).
SURGICAL TREATMENT OF HIPPOCAMPAL SCLEROSIS
Techniques for Patient Selection
The preoperative definition of HS depends on a combination of indirect methods. No single study alone is sufficient for demonstration of epileptogenicity. The use of MRI, positron emission tomography (PET), single-photon-emission computed tomography (SPECT), neuropsychological assessment, and continuous ictal and interictal video and EEG recording has resulted in 90% or better accuracy (39).
Scalp EEG (Including Spheroidal Electrodes)
MTLE with HS has a characteristic set of findings on EEG. Non-invasive scalp EEG is always performed initially. Anterior temporal spikes and sharp waves are the most common EEG, ending in patients with pathologically proven HS (40, 41, 42). Posterior and extratemporal sharp waves, if frequent or regular, suggest other pathologies (43). Bilateral independent anterior temporal spikes are not a contraindication to surgery, because most of these seizures still begin unilaterally in the medial temporal lobe (see bitemporal epilepsy below) (44,45).
Sphenoidal electrodes, though reportedly sensitive and specific for interictal activity from the mesial temporal region (40), can also record interictal activity from epileptogenic lateral temporal and orbital frontal regions (46).
Ictal discharges recorded with scalp EEG in MTLE are morphologically variable, frequently contaminated by artifact, and often delayed following clinical seizure onset. Interobserver reliability and accuracy of localization are poor, except for lateralization in patients with temporal lobe seizures (47). Temporal lobe seizures with HS can sometimes be lateralized and localized by the distinctive EEG pattern. Rhythmic theta or alpha activity within 30 seconds of the first subjective or objective indication of a seizure is strongly associated with HS and with control after resection. This pattern correctly lateralized onset in 80% of patients, as verified by intracranial recording (41) and interobserver reliability in its detection is high (48,49). Ictal onset may be bilateral and independent in up to 20% of patients (41,48,50) (see section on Bitemporal Epilepsy).
To test their use for localizing temporal lobe epileptic regions, sphenoidal electrodes were placed fluoroscopically to ensure that the recording tips were immediately below the foramen ovale (51). In a previous study (52), blindly placed sphenoidal electrodes were no better than anterior temporal electrodes in localizing yield.
Fluoroscopically placed sphenoidal electrodes were only slightly superior to anterior temporal electrodes in patients who reached class I or II Engel outcome but produced greater interrater agreement and earlier detection of ictal onset. This, the authors believed, might lead to fewer intracranial studies and less costly presurgical evaluation.
In 70% to 80% of patients with MTLE, scalp EEG, combined with other studies, will provide adequate electrical localization to accurately predict HS. Surgery is justified when there are lateralization, even without localization, of seizure onset on scalp EEG and hippocampal atrophy on MRI, with concordant unilateral temporal spikes, as long as some functional deficit confirms the mesial temporal localization and no studies provide discordant information (3).
Intracranial EEG
When localization from scalp EEG (or lateralization with other sufficient data) is lacking and other electrical documentation is needed, invasive recordings are used.
The decision to implant intracranial electrodes is not taken lightly because of the risk of hemorrhage and infection (53). The minimum number of implanted electrodes, often a combination of strip and depth electrodes, to detect the zone of seizure onset and the epileptogenic region is used (54). MRI guides stereotactic implantation of depth electrodes (55).
Before the advent of newer imaging techniques, intracranial electrodes were commonly used to evaluate MTLE. MRI can now reliably and noninvasively demonstrate HS, is highly predictive of epileptogenicity and excellent surgical outcome, and in most cases of MTLE and HS is concordant with ictal onset on scalp EEG. In light of these findings, intracranial EEG and its attendant risks can be avoided in most such patients (3).
Intracranial EEG may nevertheless be required if (i) atrophy is present on MRI without documentation of lateralized seizure onset, or with conflicting lateralization on scalp EEG, and (ii) if there is bilateral and independent temporal seizure onset.
The goals of intracranial studies for suspected MTLE are to determine lateralization (see bitemporal HS below) and to differentiate orbitofrontal, medial parietal, medial occipital, or neocortical temporal from hippocampal seizure onset. Hippocampal depth electrodes are most sensitive for documenting seizure onset in this location (Fig. 78.1) (56). These electrodes, which may be implanted from posterior to anterior to sample the length of the hippocampus, are accompanied by bilateral anterior, middle, and posterior temporal neocortical recording with either orthogonal depth electrodes or multiple subdural strips (Fig. 78.2). The electrode array is based on the clinical scenario and findings from functional imaging, scalp EEG, and MRI. A temporal grid with medial subdural strip placement is used when language mapping is needed. Because medial or orbitofrontal, parietal, or occipital seizures can mimic medial temporal seizures, additional depth or subdural electrodes may be placed in these regions when any information suggests those possibilities (53).
Recognition of normal intracranial background rhythms is essential, especially in hippocampal studies, to avoid erroneous localization (50). Physiologic electrographic activity can be seen that is not apparent on scalp EEG. Hippocampal spindles and the “third rhythm” (as coined by Niedermeyer, an α-like pattern recorded directly over the temporal neocortex predominantly in the waking and drowsy states) are unusual but normal. The absence of blocking response helps differentiate this rhythm from posterior α and μ rhythms (57).
![]() Figure 78.1 Sagittal magnetic resonance image demonstrates medial posterior depth electrode targeted to record along the length of the hippocampus with 12 individual contacts. |
In general, interictal spikes are more widespread on intracranial EEG than on scalp EEG (53,58). Multifocal spikes are common in both temporal and extratemporal epilepsy, even with a single ictal-onset region (53). Medial temporal spikes, present in more than 95% of patients with MTLE, are bilateral and independent in up to 80% (50,53,59). Although unilateral hippocampal interictal spiking (recorded from depth electrodes) has been associated with HS and good surgical outcome (60), bilateral spikes can be seen with both unilateral and bilateral HS. Ictal onset is more useful for localization.
Two patterns of seizure onset in the hippocampus have been characterized with intrahippocampal depth electrodes: an initial, periodic, high-voltage spike discharge at 1 to 2 Hz (Fig. 78.3) and a low-voltage β discharge (Fig. 78.4) (3). Seen almost exclusively in the hippocampus, the periodic pattern is related to pathologic markers of HS: it is inversely correlated with CA1 cell loss (61) and increased glial density in CA2 and CA3 (53,62). Ictal-onset morphologic features in MTLE may vary between these patterns within a single patient (63).
Seizure onset in pathologically proven HS also varies in a given patient between medial temporal locations, including hippocampus and entorhinal cortex (64), and regions of hippocampus (54). An examination of regional hippocampal atrophy by MRI found that the region of seizure onset within the hippocampus was not predicted by the distribution of maximal atrophy (54). The variability in initial seizure location and pattern suggests that MTLE (and presumably other partial epilepsies) is the product of a network of related regions rather than a single “focus.”
Evolution of the electrical activity from initial ictal changes in the hippocampus follows predictable patterns. Typically, after more than 5 seconds of the initial periodic high-voltage spike, a low-voltage rhythmic discharge of approximately 13 Hz develops in the same electrodes, decreasing in frequency as it propagates. In 60% of seizures, initial propagation is to the ipsilateral temporal neocortex, in 30% to the contralateral hippocampus, and in 10% to both structures simultaneously. Involvement of the contralateral temporal neocortex has not been seen before contralateral hippocampal onset (50). Prolonged propagation time to the contralateral hippocampus is associated with HS (65), greater neuronal loss in CA4 (66), where the main interhippocampal commissural connection begins, and there is good surgical outcome (65,67).
Frequency differences in the initial discharge can also help to distinguish hippocampal from temporal neocortical onset. Seizures beginning in the temporal neocortex have slower (4 to 10 Hz) and faster frequencies (40 to 200 Hz) than hippocampal onsets (typically 13 to 20 Hz initially or after the 1- to 3-Hz periodic pattern) (50,63,68,69), which also show more variable ictal onset frequencies.
Structural Neuroimaging
Before MRI was available, presurgical assessment frequently required intracranial EEG. HS now can be usually diagnosed by MRI non-invasively, and in combination with concordant lateralized ictal onset on scalp EEG, favorable candidates for resection are selected.
MRI protocols with coronal sections perpendicular to the long axis of the hippocampus are most effective for detecting HS (39), which appears as an increased T2-weighted signal or fluid-attenuated inversion recovery (FLAIR) in mesial temporal lobe structures (Fig. 78.5) and decreased size of the hippocampal formation, best assessed with coronal T1-weighted sequences (Fig. 78.6) (41,70, 71, 72, 73). Neuronal counts from the resected hippocampus correlate directly and significantly with quantitative measures of decreased hippocampal size (74). When neuronal cell loss is at least 50%, MRI can reliably detect HS with a sensitivity of 90% (39). Volumetric studies of atrophy and T2 relaxometry may increase the diagnostic yield and aid in detecting bilateral HS.
![]() Figure 78.3 A and B. Continuous electroencephalographic segments show periodic spike discharge at onset of seizure in the left anteromedial temporal lobe. LPT and RPT, left and right hippocampal depth electrodes; LF and RF, left and right medial frontal depth electrodes; LFP and RFP, left and right frontoparietal subdural strips; LPF, left posterior frontal subdural strip. Contacts on all electrodes are numbered from deep (1) to superficial. |
![]() Figure 78.4 A and B. Continuous electroencephalographic segments show low-voltage fast discharge at the onset of a spontaneous seizure in the left medial temporal lobe. LPT and RPT, left and right hippocampal depth electrodes; LFP, left frontoparietal subdural strip; LFT and RFT, left and right frontotemporal subdural strips. Contacts on all electrodes are numbered from deep (1) to superficial. |
Concordantly localized ictal onset on scalp EEG and hippocampal atrophy on MRI predict seizure control in more than 90% of patients undergoing temporal lobectomy (71,75, 76, 77). MRI provides the highest yield of accurate noninvasive localization for MTLE (72,73), and even by itself, hippocampal atrophy predicts excellent postoperative control in 86% of patients (71). Hippocampal atrophy on MRI is also very specific for HS (78).
The absence of hippocampal atrophy on MRI is not synonymous with the absence of HS, and in these situations, T2 signal changes can increase the yield (79). Surgical outcome without MRI-identified hippocampal atrophy is worse.
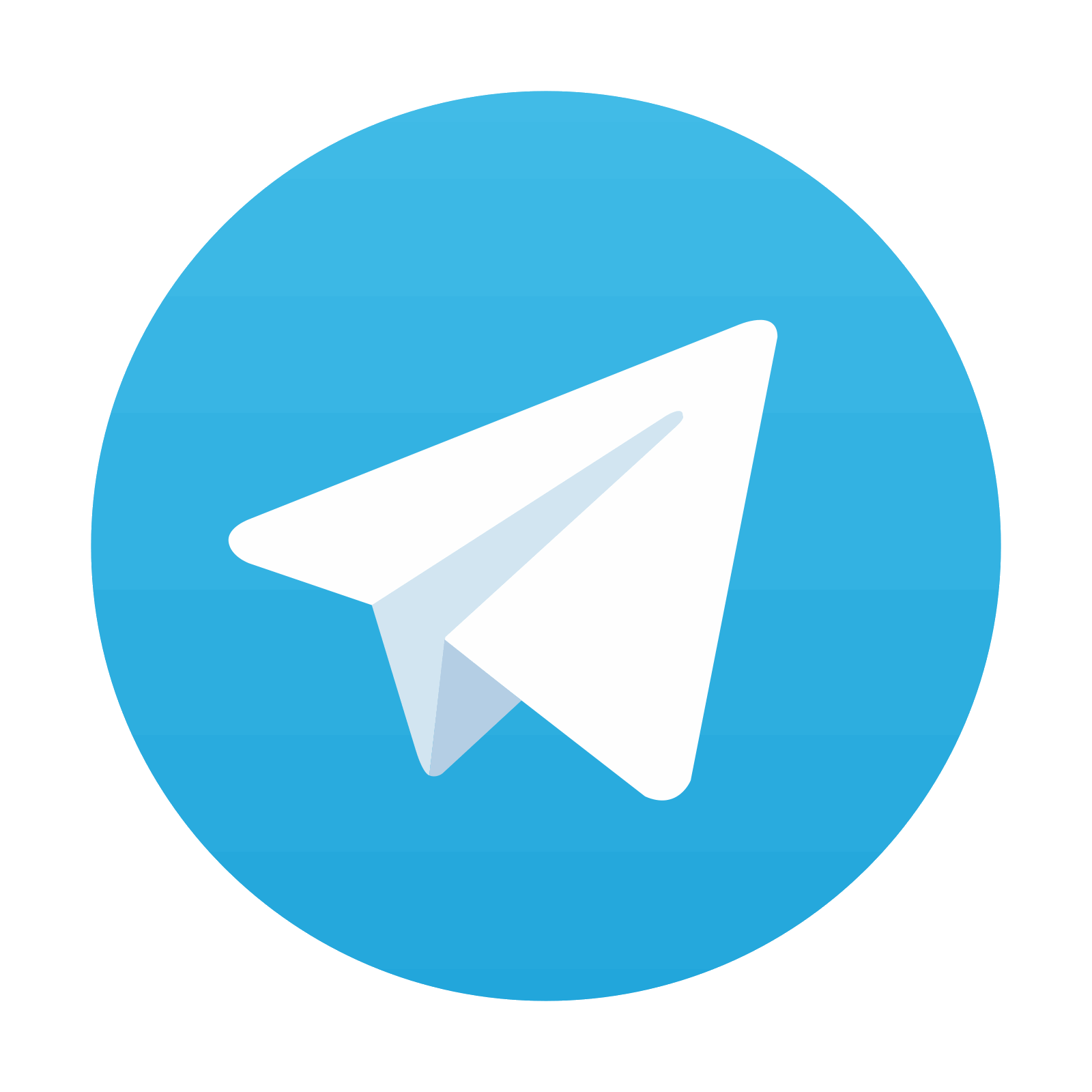
Stay updated, free articles. Join our Telegram channel
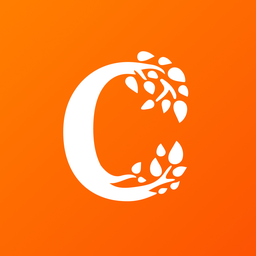
Full access? Get Clinical Tree
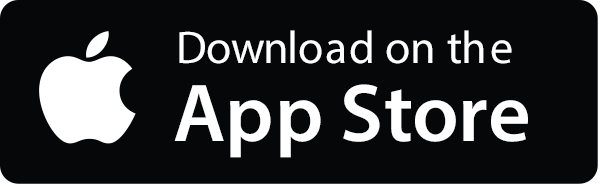
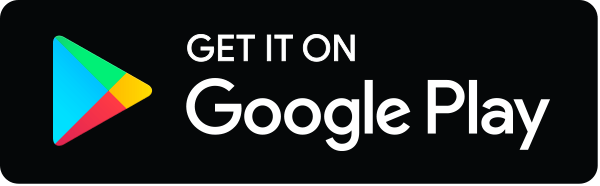