Fig. 10.1
iNSC functioning as a cell-replacement therapy and as a producer of regenerative therapeutics for stroke patients. a A patient who has an ischemic or hemorrhagic stroke (ischemic stroke shown) experiences significant brain tissue damage and loss. iNSCs could be generated from the patient’s own body by collecting adult somatic cells and reprogramming these cells using pluripotency transcription factors into induced pluripotent stem cells (iPSCs). iPSCs could then be differentiated into iNSCs and transplanted back into the patient where they would differentiate into neurons and glia that functionally integrate into the site of injury. b Transplanted iNSCs and differentiated cells have been shown to produce and may generate other regenerative and protective signaling factors including vascular endothelial growth factor (VEGF) and interleukin-10 (IL-10). (Illustration by Leah K. Schultz)
Development of Induced Pluripotent Stem Cell Technology
iPSCs are a recent discovery where mature somatic cells can be reprogrammed into pluripotent stem cells capable of differentiating into any cell type in the body through the over-expression of defined genes [24, 25]. The development of iPSC reprogramming technology resides at the convergence point of the fields of cellular reprogramming and ESCs where the conceptual framework to understand the genetic, epigenetic, and functional pluripotency networks were pioneered [26–32]. Based on prior knowledge, Yamanaka’s research team hypothesized that “the factors that play important roles in the maintenance of ES cell identity also play pivotal roles in the induction of pluripotency in somatic cells” [25]. In the mouse, embryonic fibroblasts were retrovirally transduced with 24 pluripotency-associated genes resulting in the formation of nine colonies exhibiting ESC character with cells growing in colonies and displaying a rounded morphology, large nucleoli, and high nucleus-to-cytoplasm ratio. They went on to demonstrate that only four critical factors (Pou5f1 (also known as Oct3/4), Sox2, c-Myc and Klf4) were necessary to achieve complete reprogramming of embryonic and adult fibroblast cells. iPSCs demonstrated morphology, immunoreactivity, global gene expression, and epigenetic status indicative of a pluripotent state similar to ESCs. Functional tests of plasticity demonstrated that iPSCs were capable of forming embryoid bodies (EBs; Fig. 10.2a) in vitro and teratomas in vivo consisting of all three germ layers, ectoderm, endoderm, and mesoderm. iPSCs ultimately passed the most stringent of tests and were found capable of incorporating into all tissues of chimeric mice including the germline (Fig. 10.2b) and were successful in the tetraploid complementation pluripotency assay—a test where all cells of the embryo proper are derived solely from transplanted iPSCs [25, 33–35]. The significant value of this iPSC technology for basic mouse genetics was soon recognized. However, of perhaps even greater interest was their obvious potential in human medicine for cell-replacement therapy. In 2007, the Yamanaka lab was successful in deriving the first human iPSCs using the same reprogramming genes that were successful in the generation of mouse iPSCs, thus opening the door a bit wider for personalized medicine [24].
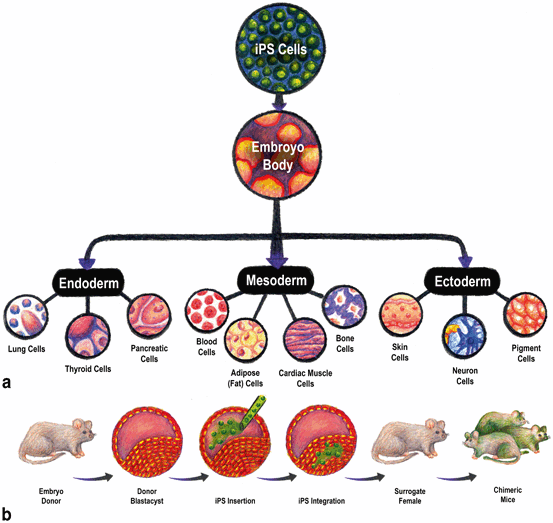
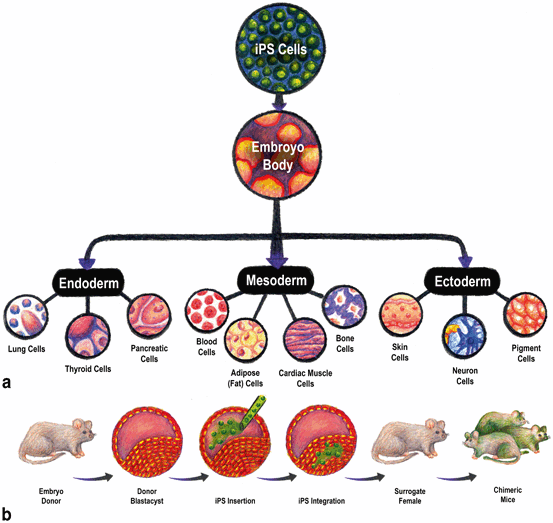
Fig. 10.2
Induced pluripotent stem cells (iPSCs) are capable of differentiating into any cell type in the body in vitro and in vivo. a To test iPSCs for their ability to form cell types of all three germ layers, a defining characteristic of iPSCs, EB differentiation is commonly used. iPSCs are induced to form large masses of cells reminiscent of developing embryos that induces spontaneous cell signaling that leads to the formation of endoderm, mesoderm, and ectoderm. Cell types representative of these lineages are commonly confirmed by immunocytochemistry of specific cell type marker expression. For example, cells can be immunostained for the neuron marker microtubule-associated protein 2 (MAP2) to identify cells of the ectoderm lineage. b To more stringently test the functional capacity of iPSCs, chimera formation is commonly performed. Embryos are collected from donor animals and are injected with iPSCs. iPSCs integrate and are transferred to a surrogate female. As the embryos develop, the integrated iPSCs are incorporated into tissues throughout the animal’s body. Chimeric offspring are then composed of cells from the donor embryo and the inserted iPSCs. (Illustration by Leah K. Schultz)
Intuitively, patients treated with their own iPSCs would be less immunogenic than those treated with allogeneic iPSCs (iPSCs derived from other patients) or ESCs; therefore, autologous iPSCs are thought to be similar to autologous human adult stem cell therapies used today in the clinic. However, there is still debate as to their immunogenicity. An early publication demonstrated that mouse iPSCs transplanted into a syngenic recipient animal, an animal that is genetically identical and transplant compatible to the mouse from which the iPSCs were derived, resulted in a T-cell-dependent immune response [36]. The researchers attributed this response to aberrant gene expression resulting from the reprogramming process. In contrast, recent studies showed little or no evidence of increased T cell proliferation or integration, antigen-specific secondary immune activity, or graft rejection in response to undifferentiated or differentiated mouse iPSCs transplanted into syngenic animals [37, 38]. These studies support the premise that autologous iPSCs may be safely transplanted into human patients without rejection; however, additional studies are needed to confirm these findings.
iPSC technology has made considerable advancements with alternative reprogramming strategies aimed at improving safety and efficiency. The initial Yamanaka lab reprogramming approach utilized spontaneous retrovirus integration of known oncogenes, including c-Myc. This random integration approach raised major concerns that insertion of genes could lead to insertional mutagenesis in addition to spontaneous reactivation of the c-Myc oncogene, which could potentially lead to tumor formation in human patients. However, recent advancements have led to novel nonintegrating approaches including minicircle DNA, modified mRNAs, and protein strategies to generate iPSCs without the need for permanent incorporation of reprogramming genes or the use of viral techniques [39–41] (Table 10.1). Efforts have also led to combinations of reprogramming factors that do not require the use of c-Myc [42]. These advances significantly improved many of the initial safety concerns that limited the potential of iPSC technology.
Table 10.1
Methods for reprogramming somatic cells to iPSCs
Vector type | Cell type | Advantage | Disadvantage | References |
---|---|---|---|---|
Integrating | Retroviral | Efficient, highly successful with numerous cells types | Genomic integration, incomplete proviral silencing and slow kinetics, formation of large numbers of partially reprogrammed colonies | |
Lentiviral | Efficient and transduces dividing and nondividing cells, highly successful with numerous cells types | Genomic integration and incomplete proviral silencing, formation of large numbers of partially reprogrammed colonies | ||
Excisable | Transposon | Efficient and integrated regions can be removed | Screening of excised lines is labor intensive | [123] |
LoxP-flanked lentiviral | Efficient and integrated regions can be removed | Exogenous genes are removed, but loxP sites are retained in the genome | [124] | |
Nonintegrating | Adenoviral | No genomic integration | Low efficiency | [125] |
Plasmid | Occasional genomic integration | Low efficiency and occasional vector genomic integration | ||
DNA free | Protein | No genomic integration, direct delivery of transcription factors and no DNA-related complications | Low efficiency, short half-life, and requirement for large quantities of pure proteins | |
Modified mRNA | No genomic integration, faster reprogramming kinetics, controllable and high efficiency | Labor Intensive | [130] |
Differentiation of Induced Pluripotent Stem Cells into Therapeutic Cells for Stroke Treatment
Stroke results in the active recruitment of endogenous NSCs in the brain leading to proliferation and migration of NSCs from the subventricular zone to the ischemic region [43–46]. This natural regenerative cell response is insufficient, however, to restore most stroke patients to their normal pre-stroke function [45, 46]. iNSCs can act as a supplemental cell source to increase the number of NSCs and the regenerative capabilities of the stroked brain. It is preferential to differentiate iPSCs into iNSCs as the direct transplantation of undifferentiated cells is likely to lead to tumor formation. Previous studies by Kawai et al. and Chen et al. showed that transplantation of undifferentiated stem cells into middle cerebral artery occlusion (MCAO) stroke models led to the development of large tumors containing cells of the ectoderm, endoderm, and mesoderm lineages [47, 48]. The differentiation of iPSCs into iNSCs has been successfully achieved using a number of different protocols originally developed for hESCs [7–10]. Oki et al. utilized a previously developed ESC approach where iPSCs were detached and grown in suspension as EBs to enhance spontaneous differentiation [9, 49]. To better direct these cells down the neural lineage, EBs were then cultured in chemically defined neural medium composed of DMEM/F12, supplemented with insulin, transferrin, progesterone, putrescine, sodium selenite, and heparin in the presence of FGF-2. Plated EBs flattened and formed small, elongated cells that generated rosette structures resembling the early neural tube. In addition to the typical neural stem cell markers SOX2 and Nestin, neural rosette cells expressed the rosette-associated transcription factors DACH1 and PLZF with apical expression of ZO-1. Neural rosettes were isolated and ultimately lost the rosette morphology and further developed into NSCs. However, these iNSCs are capable of long-term expansion, while maintaining SOX2 and Nestin expression [9]. Yuan et al. used a similar EB approach, where EBs were formed and plated but were also exposed to retinoic acid (RA) leading to the formation of rosettes [10]. Upon removal of RA, neural rosette cells detached, continued to grow in suspension and formed neural spheres. These spheres were then plated on poly-ornithine and laminin-coated dishes in serum-free media with derived cells being a homogeneous population of NSCs. Other groups have used similar systems with variations including the addition of unique growth factors, inhibitors, supporting stromal cells (e.g., PA6) and changes in timing of differentiation steps [7, 8]. Despite the variability in protocols, iNSCs are SOX1 and Nestin positive and should be capable of differentiating into multiple lineages of neurons and glia.
Intuitively it may seem that iNSCs would be the best cell type for transplantation to regenerate lost and damaged tissue. However, the plasticity of iNSCs is such that they may differentiate into any neural cell type and may differentiate into cells that are regionally incorrect. Therefore, it is of potential value to generate iPSC-derived progenitors that are regionalized. A recent report described the derivation of telencephalic progenitors, which may be valuable for treating stroke regionalized to the forebrain [21]. iPSCs were differentiated using a serum-free EB approach. Telencephalic progenitors expressed the pallial telencephalic marker PAX6 and the telencephalic marker BF1 in addition to the neural stem cell markers SOX1 and Nestin. Tornero et al. recently generated cortical neuron progenitors for the treatment of stroke noting that “Clinical and imaging data showing the distribution of ischemic cell loss underlying the most severe symptoms in stroke patients indicate that cell replacement approaches should focus on the reconstruction of damaged cortex” [23]. To produce cortically fated cells, Tornero et al. differentiated iPSCs in the presence of Wnt3A, BMP4, and cyclopamine. These cortical progenitors expressed the cortex-specific neuronal marker TBR1 and cortex markers CTIP2 and CDP (markers associated with the deeper and superficial cortex layers respectively). hESCs and iPSCs have been found to be capable of differentiating into numerous specialized neural cell types making the potential cell type options and combinations for therapeutic use numerous. The ability to transplant multiple combinations of various neural cell types to match regional-specific areas of the brain is intriguing yet adds an additional layer of complexity that will take significant consideration.
Direct Reprogramming of Fibroblasts into NSCs
Two major limitations of transplanting iNSCs into stroke patients are (1) the potential of transplanting a contaminating iPSC subpopulation that spontaneously develops into a tumor and (2) the somewhat lengthy time period it takes to generate iNSCs. Typically, it can take months to generate and sufficiently characterize iNSCs with the need to first isolate and expand the somatic cells, then reprogram the cells into iPSCs, differentiate these cells into iNSCs and then perform the necessary quality control tests on these cells prior to transplantation (e.g., cellular phenotyping, functionality assessments, karyotype analysis). A recent breakthrough in reprogramming has led to the development of technologies where somatic cells can be directly reprogrammed into neurons and NSCs without a pluripotent stem cell intermediate [50–53]. Direct neural stem cell reprogramming has been accomplished with various combinations of reprogramming factors (Table 10.2). Ring et al. was the first to show that both mouse and human fibroblasts could be reprogrammed into iNSCs with simple culture manipulations and the overexpression of the single reprogramming gene SOX2 [53]. Human cells formed clusters of SOX2 and Nestin positive cells 5 days after SOX2 retroviral transduction. These cells then underwent multiple rounds of neurosphere culture and could be maintained under standard NSC conditions. Human iNSCs were capable of differentiation into TUJ1/MAP2 + neurons, glial fibrillary acidic protein (GFAP) + astrocytes and O4/OLIG2 + oligodendrocytes. Mouse cells showed similar developmental plasticity and upon further differentiation were proven to be functionally active. Neurons derived from mouse iNSCs formed synapses marked by synapsin with patch-clamp recordings showing functional membrane properties and activity. Neither human nor mouse cells formed tumors upon transplantation into noninjured animals. Direct iNSC reprogramming provides a rapid and safe reprogramming approach with the only major limitation being the need for viral delivery and integration of reprogramming factors. Yet, building upon nonviral and nongenomic DNA-integrating approaches created for generating iPSCs, it is very likely that similar approaches can be developed for direct reprogramming of somatic cells into iNSC.
Table 10.2
Direct reprogramming into neural progenitors
Factors | Mode of reprogramming | Lineages | Species | Reference | |
---|---|---|---|---|---|
1 | ASCL1 & BRN2 & MYT1 L or ZIC1 | Lentivirus | Neuron | Mouse | [50] |
2 | ASCL1 & BRN2 & MYT1 L & NEUROD1 | Lentivirus | Neuron | Human | [51] |
3 | miR-124 and BRN2 and MYT1L | Lentivirus | Neuron | Human | [131] |
4 | SOX2 & FOXG1 & BRN2 | Lentivirus | Neurons, astrocytes and oligodendrocytes | Mouse | [52] |
5 | SOX2 & BRN4, KLF4, C-MYC (4 Factor) or with E47 (5 Factor) | Retrovirus | Neurons, astrocytes and oligodendrocytes (low) | Mouse | [132] |
6 | OCT4 & SOX2 & KLF4 & c-MYC + Significant media manipulations | Lentivirus (pre-transduced dox controlled TEFS) | Neurons and astrocytes | Mouse | [133] |
7 | ASCL1 & BRN2 & MYT1 L | Lentivirus | Neurons | Mouse | [134] |
8 | ASCL1 & NGN2 & HES1 & ID1& PAX6 & BRN2 & SOX2 & C-MYC & KLF4 | Retrovirus | Neurons, astrocytes and oligodendrocytes | Mouse | [135] |
9 | SOX2 & KLF4 & C-MYC & highly regulated OCT4 | Retrovirus | Neurons, astrocytes and oligodendrocytes | Mouse | [136] |
10 | SOX2 | Retrovirus | Neurons, astrocytes and oligodendrocytes | Mouse and human | [53] |
iNSC Transplantation into Stroke Models Leads To Promising Yet Mixed Success
iNSCs have been transplanted into mouse and rat MCAO reperfusion models with cells showing promising results [7–10, 21, 22]. However, it is difficult to compare outcomes and efficacy across studies as transplantation parameters were variable with transplant cell numbers ranging from 100,000 to 1,000,000, and timing of transplantation ranging from immediately post-reperfusion to 7 days later. The site of injection was also variable with cells being injected proximal to the lesion or in the contralateral hemisphere to the site of injury. These differences may also account for the significant amount of variability with respect to results. In general, 200,000–250,000 cells were injected 7 days later, avoiding the extreme levels of cytotoxicity immediately after stroke, into the ipsilateral lobe of stroked animals. Transplants showed survival in most studies, but the exact cell number is questionable with one study estimating 10 % cell survival [9]. Transplanted cells regularly showed differentiation into neurons, astrocytes, and oligodendrocytes with quantitative data showing higher levels of neuron differentiation than glia [9, 22]. iNSC-derived neurons showed specialization with cells differentiating into dopaminergic and gabaminergic neurons [7–9]. Functionally, whole-cell patch-clamp recordings of brain slices from iNSCs-treated mice at 5 months showed that the majority of iNSC-derived neurons tested were able to produce action potentials in response to depolarizing current and were sensitive to the voltage-gated Na+ channel blocker tetrodotoxin (TTX) and the voltage-gated K+ channel blocker tetraethylammonium (TEA) [9]. iNSC-derived neurons were also sensitive to type-A γ-aminobutyric acid (GABAA) receptor and glutamate receptor antagonists. These results and additional findings showed that iNSC-derived neurons were capable of receiving synaptic input from host neurons and functionally integrating into the neural circuitry [9].
The effect of iNSCs on endogenous tissue was inconsistent between studies. iNSCs had a protective and regenerative effect on host tissues, likely caused by paracrine signaling with the release of factors such as VEGF, as demonstrated in the Cheng et al. study [7]. They found that cell transplantation resulted in a 36 and 11 % reduction in Iba-1 + and ED1 + immune cells respectively—cells that are often associated with increased cytotoxicity. At week 8, they demonstrated a 55 % reduction in gliosis and a 17 % reduction in apoptosis. However, Oki et al. found no significant effect on immune cells (Iba-1 or ED1) or gliosis [9]. Similar studies showed no significant difference in stroke volume suggesting a minimal neuroprotective effect [9, 22].
Functional assessments again showed mixed results across studies, yet were promising. Studies showed improvements in modified neurological scores, rotarod, stepping, and staircase assessments in animals treated with iNSCs relative to nontreated controls [7–9, 21]. Animals showed mixed results with the tape removal test and failed to show significant improvement over control in the corridor, elevated body swing, and cylinder tests [9, 22]. Interestingly, the study that demonstrated the most significant functional improvement also showed the largest decrease in immune cell number, apoptosis, and gliosis [9]. This suggests a strong correlation between tissue-level improvements and positive functional outcomes.
iNSCs in Stroke May Confer Neuroprotection and Enhance Neuroplasticity and Angiogenesis Through Trophic Factor Effects
Transplantation of human iNSCs has been associated with improved functional recovery and a reduction in secondary neural degeneration in various models of ischemic stroke [7–9, 21, 23]. Most of the beneficial effects of iNSCs are observed shortly after transplantation and appear to be independent of iNSC survival suggesting that beneficial effects of iNSCs are not all attributable to cell replacement [8, 9, 21]. While the exact mechanisms through which iNSCs are able to contribute to neural recovery are not well understood, proposed mechanisms include secretion of angiogenic factors such as VEGF, neurotrophic factors such as brain-derived neurotrophic factor (BDNF) and glial cell-line-derived neurotrophic factor (GDNF), and downregulation of inflammatory mediators such as interleukin-6 (IL-6), IL-1β, and tumor necrosis factor alpha (TNF-α) [8, 9, 54]. These neuroprotective trophic factors may be secreted by the stem cells themselves or act through stimulation of endogenous protective pathways decreasing inflammation, promoting neural regeneration, angiogenesis, plasticity, and recruitment of axons from ipsilateral and contralateral hemispheres [8, 9, 55].
Routes of Cell Delivery
A variety of approaches to delivering therapeutic cells to sites of neural injury have been described [56–58]. These include intraparenchymal, intravascular, intracisternal, and intracerebroventricular injections (Fig. 10.3). To date, delivery of iNSCs for the treatment of ischemic stroke in animal models has been limited to intraparenchymal injection through transcranial approaches. Intraparenchymal approaches have also been used in several human clinical trials involving the administration of fetal porcine cells and cultured human neuronal cells to patients suffering from chronic stroke injuries [59, 60]. It is important to note, however, that intraparenchymal injections are by no means the only method of delivering cell therapy to sites of neural injury. To appreciate alternative delivery options, it is necessary to explore methods utilized with other stem cell therapies (e.g., mesenchymal stem cells, embryonic stem-cell-derived NPCs, and umbilical cord-derived cells). There are pros and cons to each delivery method, which will be described in more detail in the sections below.
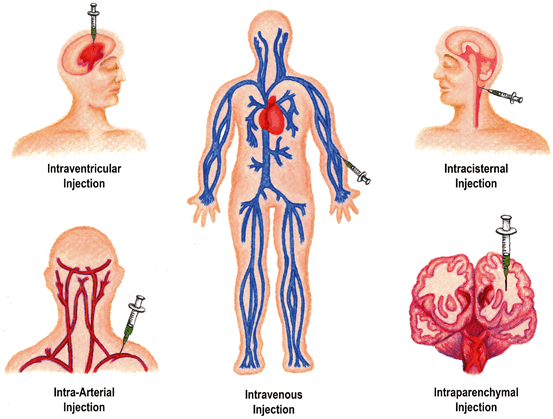
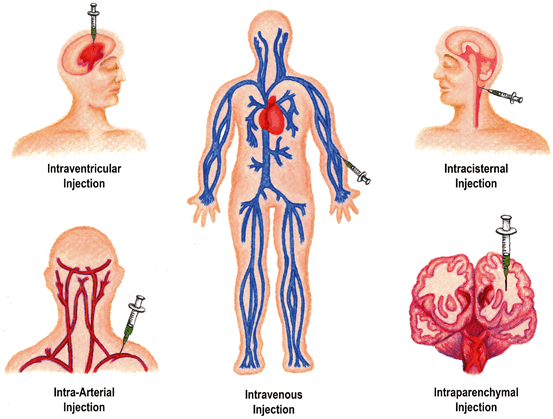
Fig. 10.3
Routes of iNSC transplantation. iNSCs can be transplanted utilizing a number of approaches: intravenous, intra-arterial, intracisternal, intraparenchymal, and intraventricular. Intravenous routes are the least invasive and least technically challenging approach with cells being injected into a peripheral vein of the patient. Successfully transplanted cell numbers are generally low with IV injections as many cells are lost to the pulmonary first-pass effect. Intra-arterial injections of iNSCs provide superior cell delivery but are associated with increased morbidity from thrombosis and hemorrhage. Intracisternal injections are moderately invasive with cells being injected into one of the subarachnoid cisterns (injection into cisterna magna shown in figure). Intraparenchymal and intraventricular injections allow more direct cell delivery but are relatively more invasive and require a transcranial approach with injection directly into the brain matter or lateral ventricles respectively. (Illustration by Leah K. Schultz)
Intraparenchymal
Intraparenchymal injections are the most commonly reported approach. This may be due to the advantages conferred by this method such as site specificity, guaranteed cell delivery to the site of injury, and direct penetration through the blood–brain barrier [58]. Unfortunately, intraparenchymal injections typically require more invasive approaches to the site of injury through burr hole craniectomies. Intraparenchymal injections may also result in more clustered, uneven distributions of cells within injured tissue relative to other cell delivery techniques such as intra-arterial (IA) injections that can accomplish a diffuse, extensive spread of cells throughout an injured region [57]. The location of the injection is dependent on the specific injury with a large proportion of MCAO models targeting injections at the site most consistently associated with infarction—the striatum [8, 9, 21]. With advanced imaging techniques such as magnetic resonance imaging (MRI), it is also possible to target injections into the peri-infarct tissue rather than into the infarct core, which may allow for improved cell survival and engraftment [61]. Implantation into either the ipsilateral or contralateral hemisphere to the injury has resulted in beneficial effects, with evidence that cells are able to migrate across midline from the contralateral hemisphere towards the site of injury [7, 62]. Injected neural progenitor cells display a predilection for injured tissues—a trait described as pathotropism [63, 64]. Ischemic injured brain tissue can secrete a variety of signals such as stromal derived factor-1 (SDF-1) and monocyte chemotactic factor 1 (MCP-1), which attract cells, including iNSCs, carrying the receptors CXCR4, CXCR7, and CCR2 [65–67]. This pathotropism will likely enhance the ability of cells to treat ischemic tissue through trophic factor signaling and improve engraftment of cells.
Intravenous
Intravenous (IV) injections are another popular route as they are generally less invasive and pose less of a technical challenge. The number of cells that need to be administered is normally greatly increased from what is permissible via intraparenchymal delivery [57]. In general, IV cell delivery results in the reduced cell engraftment and is associated with cell-uptake by systemic, nontarget organs with many of these cells being trapped in the lungs and liver [68]. The common occurrence of cells being trapped in the small vasculature of lungs is commonly referred to as the pulmonary first-pass effect [69]. Nonetheless, studies with NPCs (not of human-induced pluripotent stem cell origin) have shown that IV administration of cells in ischemic neural injury models can result in a reduction of ischemia-associated learning dysfunction, even when administration was delayed beyond the typical acute therapeutic window [70]. It is believed that these benefits arise from the production of anti-inflammatory and regenerative factors that have a systemic effect including the injured brain. In rare instances, IV delivery of cells has resulted in detectable cell engraftment within the brain [56, 71]. The ability of cells to travel from the vasculature into the brain may reflect the permeability of a compromised blood–brain barrier at the site of injury.
Intra-arterial
A means of avoiding the pulmonary first-pass effect is IA delivery of cells. This method is generally more invasive with higher patient risk for morbidity (due to hemorrhage and thrombosis) and mortality than intravenous approaches [57]. While riskier, cells administered IA have demonstrated increased migration, dissemination, and transplantation success than cells administered IV or intrathecally (IT) [57]. In a study where human mesenchymal stem cells (MSCs) were delivered IA in an MCAO rodent model, it was demonstrated that the location of transplanted cells was dependent on the timing of cell delivery [72]. Cells delivered 1 day post injury were distributed to the peri-infarct region and core of the stroke. Cells delivered on day 4 post injury demonstrated only a peri-infarct distribution. No functional improvements and only very few cells were successfully delivered when injections were administered 7 days post stroke. This would imply a limitation in the timeframe in which IA treatments are effective, although this remains to be shown with iNSCs. Timing of delivery IA may also have an impact on the phenotypic fate of transplanted NSCs with cells transplanted in the first 24 h expressing significantly more GFAP and cells transplanted at 7 and 14 days expressing more βIII-tubulin, indicating astrocyte and neuron differentiation respectively [73].
Intracerebroventricular and Intracisternal
Intracerebroventricular or intrathecal injections have also been reported as a means of delivering cells to the ischemic brain [56, 57]. These are generally associated with less patient risk than IA and intraparenchymal approaches and permit the injection of higher cell numbers. Following intra-ventricular injections, the cells are able to adhere to the walls of the ventricles and migrate through the ependymal lining into the damaged tissues, especially through the lateral versus medial walls of the ventricles [56, 57]. The exact mechanism through which the cells traverse through the ependymal lining is unknown but theories include transport through macrophage-associated regional specializations termed “fractones” [56, 74].
With intrathecal (IT) injections, cells are delivered into the cisterna magna. Again, larger cell numbers can be delivered than with intraparenchymal approaches. Unfortunately, given the flow of cerebrospinal fluid, cells can be lost to other parts of the central nervous system (CNS). In comparisons between IA, IV, and IT in rodent models, IT injections were more effective at delivering cells than IV injections, but IA was considered the superior delivery method in terms of total number of NPCs successfully delivered to the targeted tissue and the achievement of a diffuse, widespread distribution of cells within the injury [57, 75].
Delivery with an Extracellular Matrix
Survival rates of engrafted cells, regardless of the method of cell delivery, are typically low with less than half of injected cells surviving for any period of time ([76]; reviewed in [58]). One method of increasing cell survivability in the cytotoxic acute ischemic injury environment is through implantation of iNSCs with supportive extracellular matrices (ECMs). ECMs can be derived from natural materials like collagen, polyglycosaminoglycans, and ornithine/laminin or from synthetic polymers and hydrogels including polyglycolic acid (PGA), polyethylene glycol (PEG), and poly-L-lactic acid (PLLA) [77, 78]. These materials can be transformed into scaffolds with a variety of shapes and sizes with various porosities and stiffnesses to promote engraftment and recovery. In areas of severe or cystic tissue loss, as is seen in ischemic stroke, biomaterial scaffolds can act as bridging substrates to allow cell attachment and engraftment [79]. There is also suggestion that biomaterial scaffolds can play a part in inhibiting glial scar formation in some neural injury models [80]. Encapsulating scaffolds can act as barriers for grafted cells protecting from host immune rejection, while permitting signaling factors to diffuse between the graft and the injured environment [81]. As neural injuries often possess irregular boundaries, malleable and liquid substrates such as injectable hydrogels and microspheres have been particular targets of investigation [79, 82, 83]. In addition to acting as structural support, scaffolds can also be engineered to contain various growth factors, peptides, and chemical signals such as heparin and hyaluronan to promote microenvironments conducive to graft survival [78, 83–86]. Multiple studies demonstrated that ECM or NSC alone did not improve sensory motor function recovery nor decreased infarct size after focal cerebral ischemia in rodents. However, when ECM and NSCs were combined, there was a significant improvement in both functional and anatomical outcomes [82].
Despite their anticipated benefits, biomaterials can also present unique challenges including inhibition of neurite outgrowth by the scaffold [79, 87–89] and variable matrix degradation times [90]. In some cases, the scaffold may interfere with graft cell differentiation and integration [91]. For synthetic polymers, there is particular concern about harmful degradation by-products that can increase local acidity, inflammation, and tissue damage [92]. Immunoreactivity and tumorgenicity of biomaterials are also of concern, especially with undefined natural materials harvested from plant and animal sources [77]. In addition to the materials in its composition, the macro-architecture of the implants appears to play a role in the host-immune response [93]. With some scaffolds, fibrous tissue buildup and foreign-body reactions around the implant can also cause interference with tissue integration, angiogenesis, and trophic factor diffusion to and from the grafted cells ([93]; reviewed by [92]). Nonetheless, ECMs offer an exciting and viable option for improving the success of iNSC transplantation in the ischemic-stroke environment.
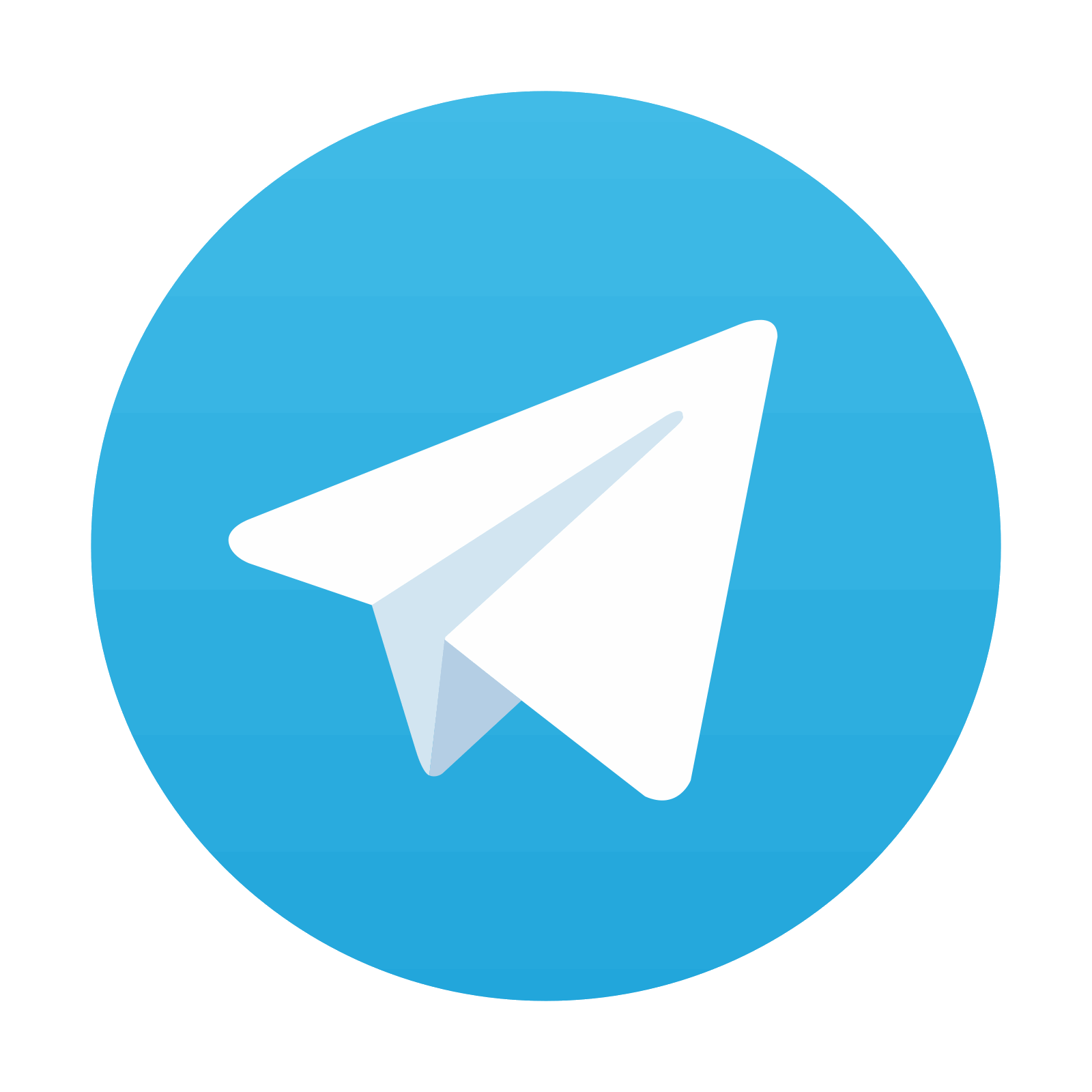
Stay updated, free articles. Join our Telegram channel
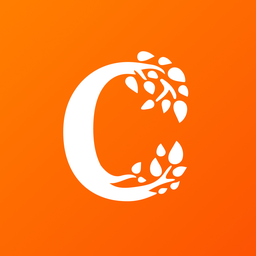
Full access? Get Clinical Tree
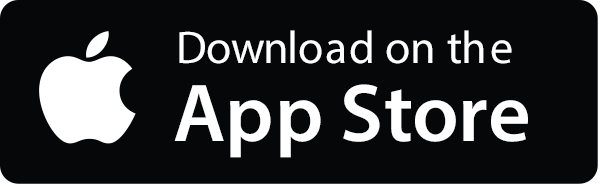
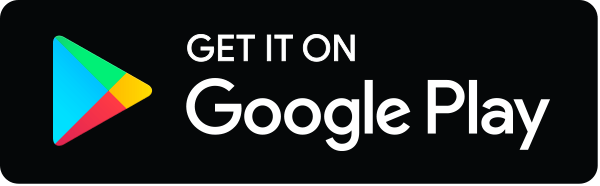