Chapter 1 Introduction to the Nervous System
The Nervous System Has Central and Peripheral Parts
The nervous system is broadly subdivided into the peripheral nervous system (PNS) and the central nervous system (CNS) (Fig. 1-1). The PNS is a collection of spinal and cranial nerves whose branches infiltrate virtually all parts of the body, conveying messages to and from the CNS. The CNS, ensconced in the skull and vertebral column, is composed of the brain and the spinal cord (Fig. 1-2). The brain itself has multiple subdivisions and is composed of the cerebrum, the cerebellum, and the brainstem. The cerebrum, in turn, is composed of the two massive cerebral hemispheres (separated from each other by the longitudinal fissure) and the diencephalon*; in an intact human brain most of the dien-cephalon is hidden from view by the massive cerebral hemispheres. The brainstem is that part of the CNS, exclusive of the cerebellum, that lies between the cerebrum and the spinal cord.
The Principal Cellular Elements of the Nervous System Are Neurons and Glial Cells
Despite the large size and widespread distribution of the nervous system, it contains only two principal categories of cells—nerve cells, or neurons, which are the information-processing and signaling elements, and glial cells, which play a variety of supporting roles. Both neurons and glial cells are present in enormous numbers. There are around 100 billion* neurons in the human nervous system and perhaps 10 times that many glial cells.
Neurons Come in a Variety of Sizes and Shapes, but All Are Variations on the Same Theme
Neurons are in the business of conveying information. They do so by a combination of electrical and chemical signaling mechanisms: electrical signals are used to convey information rapidly from one part of a neuron to another, whereas chemical messengers are typically used to carry information between neurons. Hence there are anatomically specialized zones for collecting, integrating, conducting, and transmitting information (Fig. 1-3; Table 1-1). All neurons have a cell body (soma, or perikaryon)† that supports the metabolic and synthetic needs of the rest of the neuron. Most neurons have a series of branching, tapering processes called dendrites that receive information from other neurons via synaptic contacts (or synapses) and one long, cylindrical process called an axon that conducts information away from the cell body. The axon gives rise to a series of terminal branches that form synapses on other neurons. Hence neurons are anatomically and functionally polarized, with electrical signals traveling in only one direction under ordinary physiological circumstances. (The molecular underpinnings of this anatomical and functional polarization are discussed in Chapter 7 to 9.)
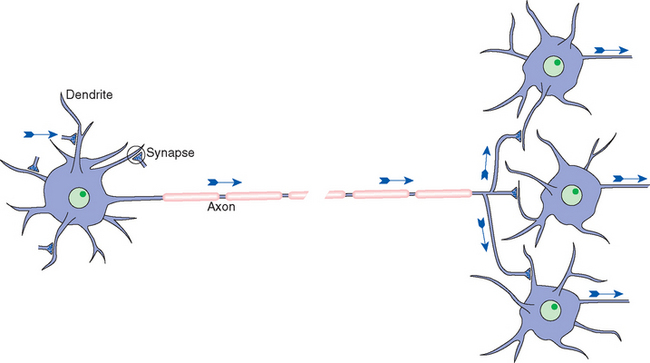
Figure 1-3 Schematic view of a typical neuron, indicating synaptic inputs to its dendrites (although other sites are possible) and information flow down its axon, reaching synaptic endings on other neurons. Information flow is unidirectional due to molecular specializations of various parts of neurons, as described in Chapters 7 and 8. The pink segments covering the axon represent the myelin sheath that coats many axons (see Figs. 1-24 and 1-30), and the gap in the axon represents a missing extent that might be as long as a meter in the longest axons.
Despite the basic similarity among all neurons, there is wide variability in the details of their shapes and sizes (Fig. 1-4). Certain aspects of somatic, dendritic, and axonal morphology give rise to a descriptive terminology for neurons. The vast majority of vertebrate neurons are multipolar, meaning that there are multiple dendritic projections from the cell body and almost always an axon as well (Fig. 1-4A to E); in many cases the pattern of the dendritic processes is characteristic of that type of neuron. Some neurons are bipolar (Fig. 1-4F) or unipolar*
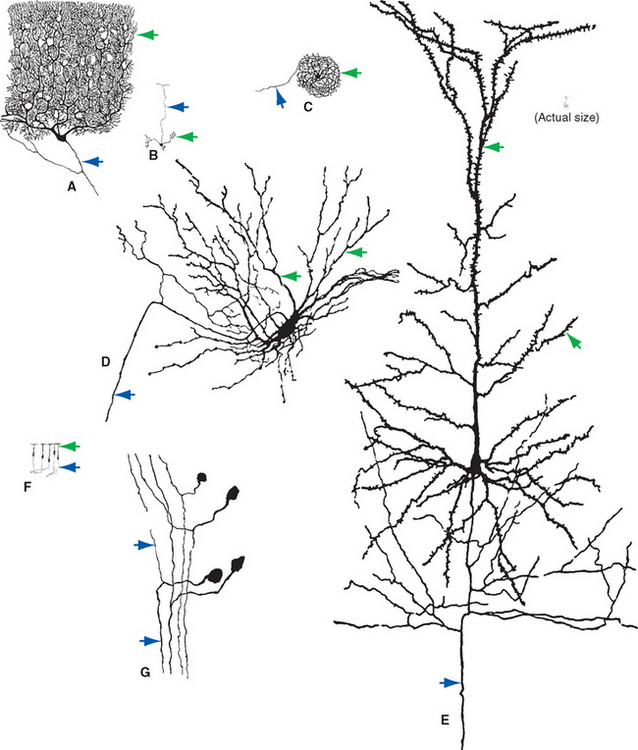
Figure 1-4 Examples of multipolar (A to E), bipolar (F), and unipolar (G) neurons, all drawn to about the same scale to demonstrate the range of neuronal sizes and shapes. All were stained by the Golgi method (see Fig. 1-14A); dendrites are indicated by green arrows, axons by blue arrows. A, Purkinje cell from the cerebellar cortex. B, Granule cell from the cerebellar cortex. C, Projection neuron from the inferior olivary nucleus. D, Spinal cord motor neuron. E, Large pyramidal neuron from the cerebral cortex. F, Olfactory receptor neurons. G, Dorsal root ganglion cells (whose processes have axonal properties along almost their entire course). The tiny inset at the upper right shows the actual size of the pyramidal neuron.
(Modified from Ramón y Cajal S: Histologie du systãme nerveux de l’homme et des vertébrés, Paris, 1909, 1911, Maloine.)
(Fig. 1-4G), having two processes or only one, respectively. There is a broad spectrum of not only neuronal shapes but also neuronal sizes. Cell bodies range from about 5 to 100 μm in diameter. Many axons are short, only a millimeter or so in length; but some, like those that extend from the cerebral cortex to the sacral spinal cord, measure a meter or more.† For many years, the major technique available for studying the shapes and sizes of neurons was Golgi staining, a method that* infiltrates all the processes of a small percentage of neurons with heavy metals, causing them to stand out from an unstained or counterstained background (see Figs. 1-4 and 1-14A). More recently, however, a variety of methods relying on microinjection or immunocytochemical techniques have become available (Box 1-1). These now make it possible to correlate the structure of an individual neuron with aspects of its function.
BOX 1-1 Making the Morphology of Individual Neurons Visible
One drawback of Golgi staining is that it stains a subset of neurons indiscriminately (Fig. 1-14A), revealing relatively little about the function of an individual cell. The last few decades have seen the development of increasingly sophisticated techniques for demonstrating the morphology of functionally identified neurons.
A mainstay in the study of the electrophysiological properties of individual neurons has been the use of micropipette electrodes that either impale single neurons or attach to their surfaces (see Chapter 7). The same electrodes can be used as tiny hypodermic needles to inject a dye or marker substance, allowing study of the anatomy of the same neuron (Fig. 1-5). Such injections can reveal morphological detail comparable to that shown by Golgi staining (Figs. 1-5 and 1-6).
Different classes of neurons also have chemically different interiors, and it is possible to make labeled antibodies that demonstrate some of these differences (Fig. 1-6D). Correlations of neuronal morphology and location with neurotransmitter content have been particularly instructive. For example, neurons that use norepinephrine as the chemical transmitter at their synapses contain this substance throughout their axons and cell bodies. Appropriate fixation and processing cause these neurons to be fluorescent. Alternatively, it is possible to make a labeled antibody to an enzyme involved in the formation of a neurotransmitter or a labeled antibody to a receptor for a given transmitter. Such methods have made it possible to map out “chemically coded” neural pathways (see Chapter 11). Methods for studying neurotransmitter content and electrophysiological properties can be combined to produce particularly elegant structure-function correlations (Fig. 1-6).
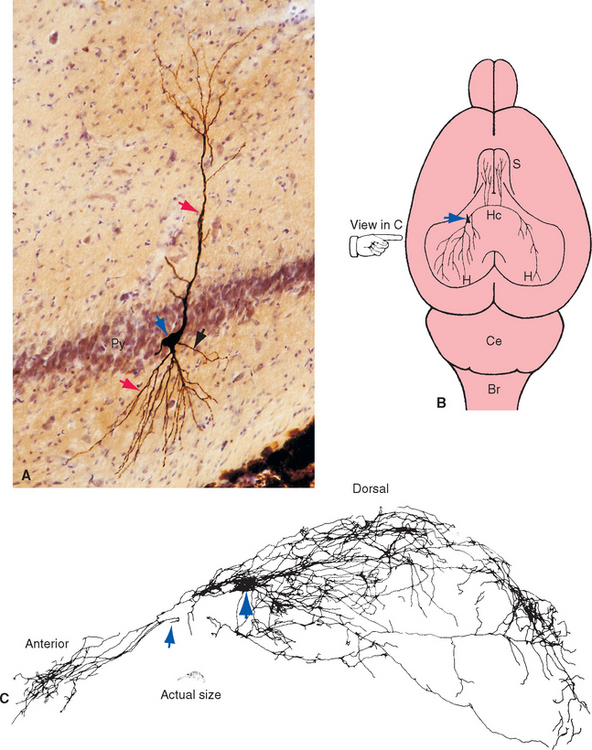
Figure 1-5 Morphology of an individual neuron revealed by injection of a marker substance from an intracellular recording electrode. This study shows the striking degree of anatomical detail that can be demonstrated using this technique. Although we tend to draw neurons as fairly simple cells with a single axon going from one place to another, they are in reality far more complicated. A, Pyramidal neuron from the hippocampus of a rat, injected with horseradish peroxidase. The marker was subsequently visualized using an immunocytochemical technique, and the cell body (blue arrow) of the injected neuron stands out from the neighboring neurons in the pyramidal cell layer (Py); dendrites (red arrows) and a single axon (black arrow) emerge from the cell body. B, Examining stained processes of this neuron in many adjacent sections led to the conclusion that the axon has a long, complex set of branches, as shown schematically in this view of the dorsal surface of a rat’s brain. The hippocampus (H) is a specialized area of cerebral cortex buried within the cerebral hemisphere (see Chapter 24). The labeled hippocampal pyramidal neuron (blue arrow) sends axonal branches to the septal nuclei (S) bilaterally, to the hippocampus in which the neuron resides, and to the opposite hippocampus by way of the hippocampal commissure (Hc). C, Drawing of a reconstruction of the neuron and its branches in the injected hemisphere, compiled from numerous adjacent parasagittal sections. The view is from the side, as indicated by the hand in B. At this magnification the cell body is a small structure (large blue arrow) surrounded by dendrites. The axon branches extensively and sends projections both anteriorly and posteriorly. In this reconstruction, it ends at the point (small blue arrow) where one of its branches prepares to cross the midline and project to the contralateral hemisphere. Br, brainstem; Ce, cerebellum.
(Modified from Tamamaki N, Watanabe K, Nojyo Y: Brain Res 307:336, 1984.)
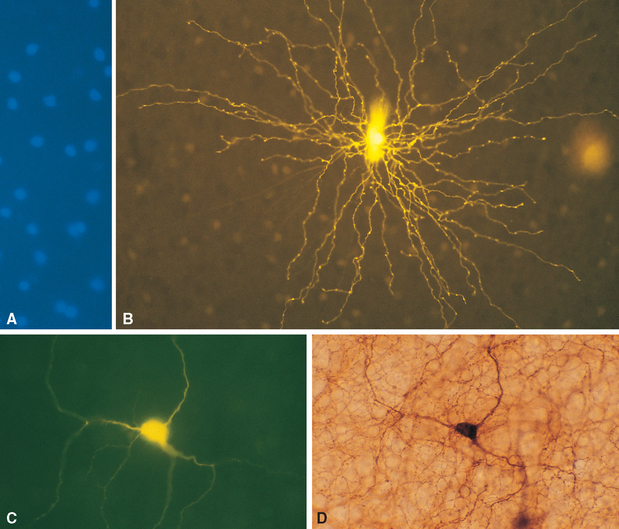
Figure 1-6 Combined use of neurotransmitter identification techniques and intracellular injection of dye. A and B, One subset of retinal amacrine cells (see Chapter 17) uses serotonin as a neurotransmitter. These neurons accumulate serotonin from the surrounding medium and also accumulate certain analogs of serotonin. A, A fluorescent analog (5,7-dihydroxytryptamine) was applied to a living, flat-mounted rabbit retina, which was then viewed using ultraviolet illumination. Serotonin-accumulating amacrine cells fluoresce blue under these conditions, allowing chemically identified neurons to be impaled by dye-filled micropipette electrodes. B, Serotonin-containing amacrine cell injected with a fluorescent dye (Lucifer yellow). Details of the long, mostly unbranched dendrites of this neuron are readily apparent. C and D, Another subset of retinal amacrine cells uses dopamine as a neurotransmitter. C, One such amacrine cell was first injected with a fluorescent dye (Lucifer yellow). D, The same area of retina was then stained with an antibody to tyrosine hydroxylase (an enzyme involved in the synthesis of dopamine). The obvious correspondence between the two images indicates that the injected amacrine cell manufactures dopamine.
(A and B, courtesy Dr. David I. Vaney, National Vision Research Institute of Australia. C and D, courtesy Dr. Dennis M. Dacey, University of Washington School of Medicine.)
Neurons can also be classified according to their connections. Sensory neurons either are directly sensitive to various stimuli (such as touch or temperature changes) or receive direct connections from nonneuronal receptor cells. Motor neurons end directly on muscles, glands, or other neurons in PNS ganglia. Most sensory and motor neurons live partly in the PNS and partly in the CNS (see Fig. 1-8), whereas almost all other neurons reside entirely in the CNS and interconnect other neurons. Some are local interneurons and have all their processes confined to a single small area of the CNS. Others are projection neurons, with long axons connecting different areas, such as a neuron in the cerebral cortex whose axon reaches the spinal cord. In a strict sense, the human nervous system is composed almost entirely of interneurons and projection neurons: there are at most 20 million sensory fibers in all the spinal and cranial nerves combined and no more than a few million motor neurons. Even taking into account the autonomic neurons that innervate muscles and glands (see Chapter 10), more than 99% of our neurons are interneurons or projection neurons. However, the words sensory and motor are often used in a much broader sense to refer to cells and axons that carry information related to sensory stimuli and to the generation of responses, respectively.
Neuronal Cell Bodies and Axons Are Largely Segregated within the Nervous System
For the most part, the CNS is easily divisible into gray matter and white matter (Figs. 1-7 and 1-8). Gray matter refers to areas where there is a preponderance of cell bodies and dendrites; in life, however, it is actually a pinkish gray color because of its abundant blood supply. White matter refers to areas where there is a preponderance of axons; many axons have a myelin sheath (described later in this chapter) that is mostly lipid and therefore has a fatty, white appearance.
Specific areas of gray matter are often called nuclei,* particularly if the contained cell bodies are functionally related to one another. An area where gray matter forms a layered surface that covers some part of the CNS is referred to as a cortex. The cerebral and cerebellar cortices are the two most prominent examples. Occasionally, descriptive names are used for particular areas of gray matter (e.g., the putamen, a nucleus in the cerebral hemisphere named for its shape and location), but these are relatively infrequent.
In contrast, subdivisions of white matter (i.e., collections of axons) go by a bewildering variety of names,† such as fasciculus, funiculus, lemniscus, peduncle, and, most commonly, tract. Many tracts have two-part names that provide some free information about the nature of the tract; the first part of the name refers to the location of the neuronal cell bodies from which these axons originate, and the second part refers to the site where they terminate. Thus a spinocerebellar tract is a collection of axons with cell bodies in the spinal cord and synaptic endings in the cerebellum.
The spinal cord provides a reasonably clear example of the separation of neural tissue into gray matter and white matter (Fig. 1-8). Sensory axons, whose unipolar cell bodies are located in the dorsal root ganglia of spinal nerves, enter the spinal cord and divide into a large number of branches, most of which terminate on neuronal processes in the spinal gray matter. Motor axons, whose multipolar cell bodies are located in the spinal gray matter, leave the spinal cord and enter spinal nerves. The white matter contains long descending tracts (from the brainstem and cerebrum), long ascending tracts (to the brainstem, cerebellum, and cerebrum), and local axons interconnecting different spinal levels. The gray matter, in contrast, contains motor neurons, the endings of incoming sensory axons and long descending tracts, local interneurons, and projection neurons whose axons enter long ascending tracts. This division into white and gray matter is rarely absolute; for example, axons in long descending tracts obviously must pass through some gray matter before reaching their targets.
Peripheral nerves are, for most of their courses, collections of axons on their way to or from places such as skin, muscle, or internal organs, accompanied by glial and connective tissue sheaths (see Fig. 9-19). Many of these axons have cell bodies that also reside in the PNS, and these somata are typically clustered in ganglia at predictable sites along the nerve (Fig. 1-8).
Neuronal Organelles Are Distributed in a Pattern That Supports Neuronal Function
Neurons need mechanisms to deal not only with their electrical and chemical signaling functions but also with other consequences of their extended anatomy. A large neuron with a long axon (e.g., one of the neurons shown in Fig. 1-4A, D, and E) may have 99% of its cytoplasm in the axon, much of it many centimeters away from the cell body; hence its single nucleus and associated synthetic apparatus must have efficient mechanisms for communicating with distant parts of its appendages. In addition, brains have no bones, but neurons have long, delicate processes, so there is a need for mechanical stabilization that can be met only partially by the external suspension mechanisms described in Chapters 4 and 5. To address these issues, neurons, like other cells, contain a nucleus and an assortment of organelles—mitochondria, endoplasmic reticulum, Golgi apparatus, and cytoskeletal elements—but the abundance and configuration of these organelles in different parts of a neuron reflect the function of each of these parts.
Neuronal Cell Bodies Synthesize Macromolecules
The neuronal cell body is the site of synthesis of nearly all the neuron’s enzymes, structural proteins, membrane components, and organelles, as well as some of its chemical messengers. Its structure (Fig. 1-9) reflects this function. The nucleus is large and pale-staining, with most of its chromatin dispersed and available for transcription; it contains one or more prominent nucleoli, which are actively involved in the transcription of ribosomal RNA. The cytoplasm contains abundant rough endoplasmic reticulum and free ribosomes for protein synthesis, together with stacks of Golgi cisternae for further processing and packaging of synthesized proteins. Many mitochondria are also present to meet the energy requirements of continuous, very active protein synthesis.
Ribosomes, whether studding the surface of the rough endoplasmic reticulum or free in the cytoplasm between the cisternae, are stained intensely by basic dyes, appearing by light microscopy as clumps called Nissl bodies or Nissl substance (Fig. 1-10). Nissl bodies are particularly prominent in large neurons, a consequence of the large total volume of cytoplasm contained in their processes, and they appear in characteristic configurations in different neuronal types.
The organelles just described are embedded in a network of three kinds of filamentous protein polymers that extend throughout the neuron and its processes, collectively constituting the neuronal cytoskeleton. Microtubules are cylindrical assemblies, about 25 nm in diameter, of 13 strands (protofilaments) of protein arranged around a hollow core. Each protofilament is a polymer of the protein tubulin; an assortment of additional proteins associated with the microtubules links them to one another, to other cytoskeletal elements, and to various organelles as they travel toward or away from the cell body. Neurofilaments, the neuron’s version of the intermediate filaments found in most cells, are multiply twisted, ropelike assemblies of strands of polymers involving at least three different proteins from the cytokeratin family. Neurofilaments are about 10 nm in diameter, much too small to be seen under the light microscope, but they aggregate in response to certain chemical fixatives. When silver stains are applied, such aggregates can be visualized as neurofibrils (Fig. 1-11). Finally, microfilaments, the thinnest cytoskeletal element (7 nm), are twisted pairs of actin filaments. All three kinds of cytoskeletal elements contribute to maintaining the shape of the neuron. Microtubules also serve as the substrate along which organelles are transported through neuronal processes (as described in more detail later in the chapter). Microfilaments are important for anchoring membrane molecules in place (e.g., receptor molecules at synapses), for shuttling things to and from the cell membrane, and for movement of the advancing tip of growing axons.
< div class='tao-gold-member'>
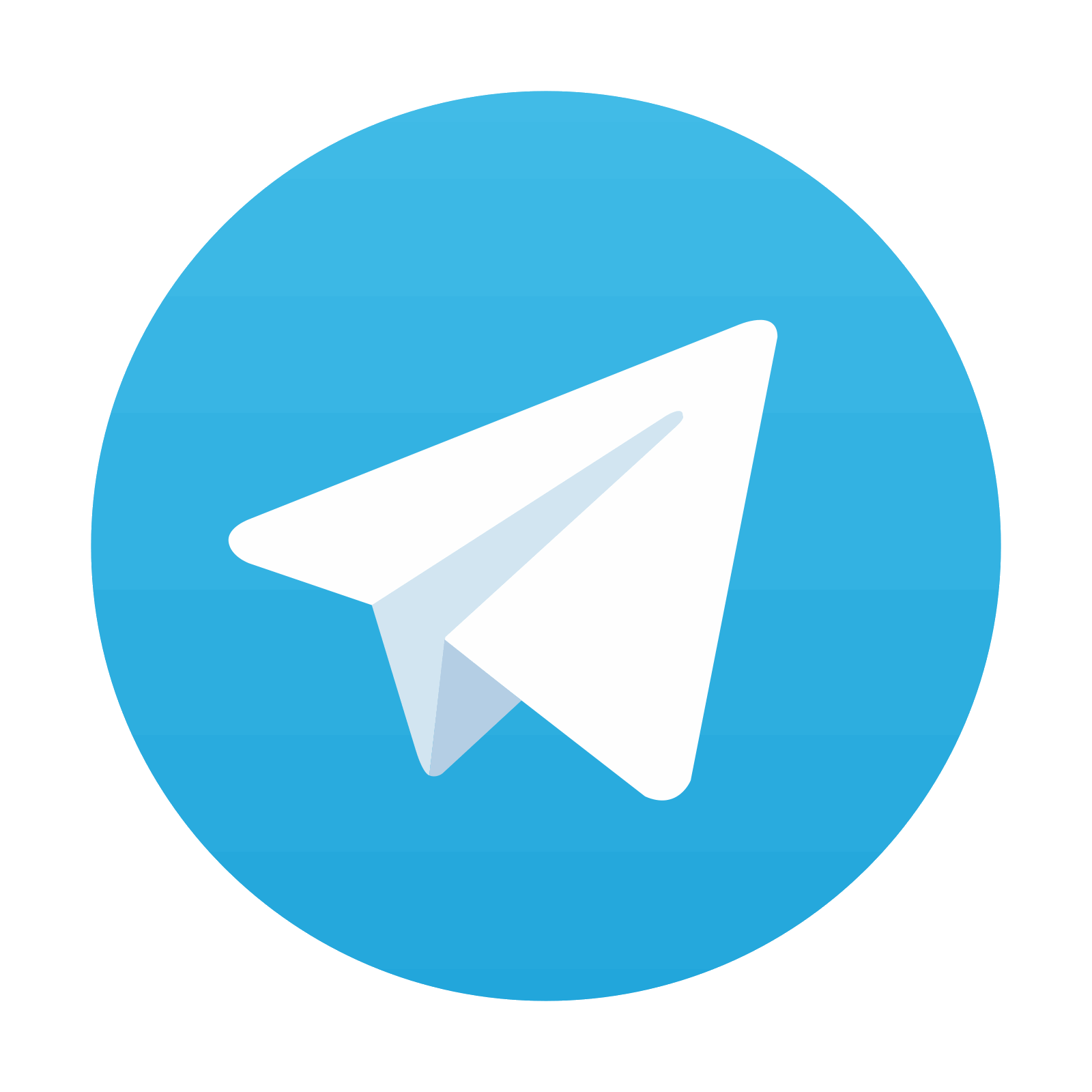
Stay updated, free articles. Join our Telegram channel
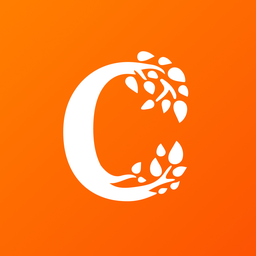
Full access? Get Clinical Tree
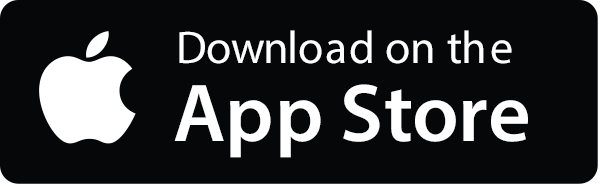
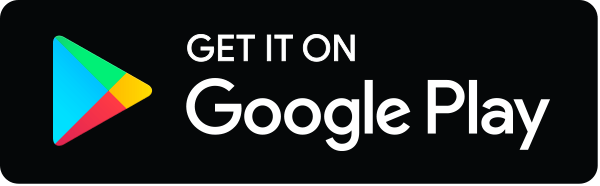