Chapter 8 Synaptic Transmission between Neurons
Early in the last century, Ramón y Cajal and others used the Golgi stain to demonstrate that the nervous system is a collection of individual neurons (e.g., see Fig. 1-14A) rather than a vast syncytial network, as some had alleged. An obvious corollary of this demonstration is that neurons must have mechanisms by which they communicate with one another. Although there are some instances in which neurons are directly coupled, allowing ionic currents to flow from one into another (discussed at the end of this chapter), in most cases neurons communicate with one another by releasing neuroactive chemical transmitters, typically at specialized sites called synapses.*
The first insights into how synapses between neurons might work came from studies of the neuromuscular junction. There the endings of motor neurons release a small-molecule transmitter (acetylcholine), which diffuses across the cleft between neuronal ending and muscle fiber, attaches to receptor molecules in the muscle fiber membrane, and initiates permeability changes and consequent rapid depolarization (Fig. 8-1; see also Figs. 8-10 and 8-11). The depolarization is short-lived because an enzyme (acetylcholinesterase) simultaneously competes for acetylcholine and hydrolyzes it. It is now apparent that the neuromuscular junction is representative of only one type of synaptic interaction. Dozens of neurotransmitters have been described to date. Some are small molecules such as acetylcholine (see Figs. 8-18, 8-19, and 8-21 to 8-23), whereas others are larger peptide molecules or diffusible gases; some produce brief depolarizing or hyperpolarizing changes in membrane potential, whereas others produce prolonged potential changes (Fig. 8-2) or changes in membrane properties that last for days or longer.*
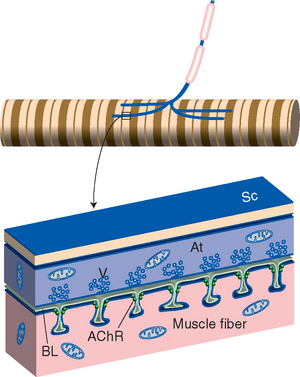
Figure 8-1 General arrangement of a neuromuscular junction (see Fig. 8-11 for a scanning electron micrograph of a real junction). A motor axon loses its myelin sheath and divides into several terminal branches (At) covered by processes of Schwann cells (Sc; not indicated in the upper drawing). Each terminal contains a series of clusters of vesicles (V) filled with acetylcholine. Invasion of a terminal by an action potential causes some of the vesicles to merge with the terminal membrane and dump their contents into the cleft between the terminal and the muscle fiber. The liberated acetylcholine diffuses across the cleft and through the basal lamina (BL) and reaches acetylcholine receptor molecules (AChR) at the entrance to troughs in the muscle surface across from each vesicle cluster. These acetylcholine receptors are ligand-gated cation channels, and binding acetylcholine causes depolarization of the muscle fiber (see Fig. 8-10). The action of acetylcholine is temporally limited by the enzyme acetylcholinesterase, associated with the basal lamina, which competes for and hydrolyzes acetylcholine.
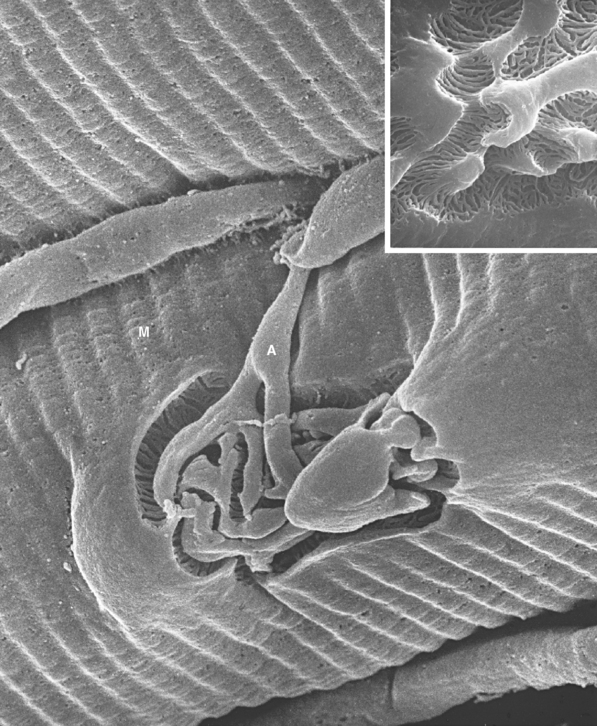
Figure 8-11 Three-dimensional structure of a neuromuscular junction. This scanning electron micrograph shows a motor axon (A) approaching a muscle fiber (M) in a calf muscle (peroneus longus) of a hamster. The axon divides into a series of terminal branches that occupy grooves in the surface of the muscle fiber. Removing these branches from a similar neuromuscular junction reveals a series of troughs traversing the grooves, each corresponding to one of the troughs in Figure 8-1 and containing acetylcholine receptors. The actual size of the end plate is about 7 × 12 μm.
(From Pannese E: Neurocytology: fine structure of neurons, nerve processes, and neuroglial cells, New York, 1994, Thieme Medical Publishers.)
There Are Five Steps in Conventional Chemical Synaptic Transmission
Chemical synapses come in a variety of shapes and sizes, but they all include as their essential components a presynaptic ending and a postsynaptic element, separated by a 10- to 20-nm synaptic cleft (see Fig. 1-19). The presynaptic elements are usually either terminal expansions of axons (Fig. 8-2) or expansions of axons as they pass by other neuronal elements (referred to as boutons terminaux and boutons en passage, respectively—French for “terminal buttons” and “buttons along the way”). In some instances, however, dendrites or even parts of neuronal cell bodies can be presynaptic elements. Similarly, the postsynaptic element is usually part of the surface of a dendrite, but alternatively, it can be located on a cell body, axon initial segment, or another synaptic terminal (Fig. 8-3; see also Fig. 1-20). All these locations have functional implications, as described later in this chapter.
Neurotransmitters Are Synthesized in Presynaptic Endings and in Neuronal Cell Bodies
Nearly all known or suspected neurotransmitters fall into one of two general categories: some are small molecules such as amines or amino acids, and the others are peptides (neuropeptides). Small-molecule transmitters are synthesized in presynaptic cytoplasm, using locally available substrates (e.g., acetate and choline) and soluble enzymes that arrive by slow axonal transport (Fig. 8-4A). Making peptide transmitters, in contrast, requires the protein synthesis machinery that resides in the neuronal cell body. Neuropeptides start out there as larger precursor proteins that are packed into vesicles and dispatched by fast axonal transport to synaptic endings. Along the way, the precursors are cleaved and processed into the final, biologically active neuropeptides (Fig. 8-4B).
Neurotransmitters Are Packaged into Synaptic Vesicles before Release
Synaptic vesicles are the units of currency at chemical synapses, the sites where neurotransmitters are packaged, concentrated, and protected from catabolism while awaiting release. All presynaptic endings contain small vesicles (about 40 nm), and many also contain less numerous but larger vesicles (≥100 nm). Depending on the preparation conditions used for electron microscopy, some of the small vesicles may appear dark, and others may look clear and either round or flattened; each large vesicle contains a dark core (Fig. 8-5). These differences in appearance correspond to differences in neurotransmitter content. For example, endings with clear, flattened vesicles are usually inhibitory.
Small synaptic vesicles contain small-molecule transmitters, concentrated to levels far beyond that found in the cytoplasm by specific transporters in the vesicle walls. Large dense-core vesicles contain neuropeptides at lower concentrations and may contain one or more small-molecule transmitters as well. The two kinds of vesicle, like the two kinds of neurotransmitter, are manufactured and recycled differently (Fig. 8-4). Small-molecule transmitters are synthesized by cytoplasmic enzymes and can therefore be manufactured and packaged for release in individual synaptic endings. Small synaptic vesicles can therefore be recycled entirely within a presynaptic ending, whereas large dense-core vesicles must be created anew in the cell body.
Presynaptic Endings Release Neurotransmitters into the Synaptic Cleft
Release of neurotransmitter is a Ca2+-mediated secretory process. Each presynaptic density, or active zone, contains an abundance of voltage-gated Ca2+ channels, together with anchoring sites for a cluster of small vesicles that are held there (“docked”) by a Ca2+-sensitive system of proteins. Depolarization of the presynaptic terminal, such as by propagation of an action potential down the axon and subsequent electrotonic spread into the terminal, causes opening of the voltage-gated Ca2+ channels. Because the free intracellular Ca2+ concentration is only about 10−7 M, Ca2+ ions flow in through these channels and momentarily elevate the Ca2+ concentration near the active zone by as much as 1000-fold. During the brief period before the excess Ca2+ diffuses away or is sequestered, a vesicle docked nearby may fuse with the presynaptic membrane and discharge its contents into the synaptic cleft in a process called exocytosis (Fig. 8-6A). The whole process, from the arrival of an action potential to the release of a small vesicle’s contents, can take less than 100 μsec. Because the large, dense-cored vesicles are not located adjacent to active zones, repetitive action potentials, additional Ca2+ entry, and more time (10s of milliseconds) are typically required for their exocytosis (Fig. 8-6B). This exocytotic addition of membrane clearly cannot continue for very long, or else presynaptic endings would expand continuously. In fact, vesicle membranes are taken back up (by endocytosis), and those used for small-molecule transmitters can be recycled in less than a minute (Fig. 8-4A).
Neurotransmitters Diffuse across the Synaptic Cleft and Bind to Postsynaptic Receptors
Neurotransmitters released from small vesicles find postsynaptic receptor molecules waiting for them directly across the synaptic cleft (Fig. 8-7). For this reason, it takes the contents of such vesicles very little time to exert their effects. The total synaptic delay from presynaptic action potential to postsynaptic effect can be less than 200 μsec. The contents of large vesicles, in contrast, take longer to be released and often diffuse to receptors relatively far away (see Fig. 8-9), so their effects develop more slowly.
Neurotransmitter Action Is Terminated by Uptake, Degradation, or Diffusion
Neurotransmitter molecules need to be removed quickly once they have had a chance to bind to receptors, so that the postsynaptic membrane will be prepared for subsequent releases of transmitter. This is accomplished by virtually every means imaginable (Fig. 8-8). Binding of neurotransmitter and receptor is a reversible event, so receptors and removal mechanisms compete for transmitter. Some transmitter simply diffuses away, but this is too slow a process to be the principal mechanism. In most synapses, transmitter is reabsorbed into the presynaptic ending or taken up by neighboring glial cells or even by the postsynaptic process. In others, enzymes in the synaptic cleft degrade free transmitter. Reabsorbed transmitters or their metabolic products, like vesicle membranes, are often recycled for use in a subsequent synaptic event.
Different kinds of neurotransmitters have different preferred mechanisms of removal. For example, serotonin, norepinephrine, and dopamine are transported rapidly back into the presynaptic ending for repackaging in synaptic vesicles. Acetylcholine, in contrast, is split into acetate and choline by acetylcholinesterase in the synaptic cleft; the choline is then transported back into the presynaptic ending and used for the synthesis of more acetylcholine. Neuropeptides are either degraded by extracellular peptidases or swallowed up by the postsynaptic cell while still attached to their receptors (Fig. 8-9).
Synaptic Transmission Can Be Rapid and Point-to-Point, or Slow and Often Diffuse
Postsynaptic events in response to transmitter-receptor binding fall into two general categories—fast and slow. Some postsynaptic responses involve electrically silent metabolic or membrane changes, but most involve depolarizing or hyperpolarizing potential changes across the postsynaptic membrane. A depolarizing response brings the postsynaptic element closer to its threshold for firing an action potential and so is referred to as an excitatory postsynaptic potential (or EPSP). Conversely, a hyperpolarizing response moves the membrane away from the threshold and so is referred to as an inhibitory postsynaptic potential (or IPSP). Hence there are fast and slow EPSPs, and fast and slow IPSPs.
Fast synaptic potentials are used for point-to-point transfer of specific bits of information—for example, by motor neurons projecting to particular muscle fibers or by tract cells projecting to particular parts of the thalamus (e.g., see Fig. 10-19). Slow synaptic potentials are sometimes used in this way too, but many are generated by diffusely projecting neurons with hundreds of branches that regulate the overall activity level of broad expanses of the nervous system (e.g., see Figs. 11-24 and Fig. 11-26 to 11-28).
Rapid Synaptic Transmission Involves Transmitter-Gated Ion Channels
Early studies of synaptic transmission demonstrated that a single action potential in the motor nerve ending at a neuromuscular junction causes a large but brief EPSP,* sufficient to cause an action potential and contraction in the muscle fiber (Fig. 8-10B). Closer analysis revealed small, brief depolarizing events in the postjunctional muscle membrane during the periods between action potentials (Fig. 8-10A), each corresponding to a brief period of channel opening and depolarizing current flow. We now know that each of these small depolarizing events is the response of the postsynaptic membrane to the spontaneous release of one synaptic vesicle. Each vesicle contains about 10,000 acetylcholine molecules, which diffuse across the synaptic cleft and bind briefly to acetylcholine receptors, which themselves are ligand-gated ion channels permeable to both Na+ and K+ ions. Acetylcholinesterase competes with the receptors for the released acetylcholine, so the channels stay open for only a millisecond or two. During this time, they allow a current flow that tries to move the membrane potential to a value around 0 mv (between the Na+ and K+ equilibrium potentials; see Appendix 7B, Equation B-7). Because 2 msec is considerably less than the time constant of the muscle membrane, the membrane potential never reaches 0 mv. Instead, it rises rapidly at the beginning of the postsynaptic current flow and then decays slowly at the end of the current flow (Fig. 8-10A). Entry of depolarizing current is localized to the site of the receptors, so the postsynaptic potential decays electrotonically (Fig. 8-10C and D). However, the presynaptic ending at each neuromuscular junction contains upwards of 1000 active zones (Figs. 8-7 and 8-11), so a single action potential in the motor axon causes the nearly simultaneous release of hundreds of vesicles full of acetylcholine at closely spaced sites, which in turn causes the large EPSP normally seen (Fig. 8-9B).
The basic elements of neuromuscular transmission have proved to be generally true of fast synaptic transmission throughout the nervous system (Fig. 8-12A). Depolarization-induced Ca2+ entry causes the release of one or more packets of neurotransmitter, called quanta, each of which is generally assumed to be the contents of a single vesicle docked at an active zone. The neurotransmitter then diffuses across the synaptic cleft and binds transiently to a transmitter-gated (i.e., ligand-gated) ion channel. The selectivity of the channel determines the postsynaptic effect. Opening channels selective for monovalent cations, as in the example of the neuromuscular junction, causes an EPSP. Opening channels selective for Cl−, in contrast, moves the membrane potential toward the Cl− equilibrium potential; this is the most common basis of fast IPSPs in the central nervous system (CNS). The selective concentration of receptors on the postsynaptic membrane ensures that fast postsynaptic potentials are localized spatially. Transmitter-gated ion channels are also referred to as ionotropic receptors (from the same Greek word that gave rise to tropism, a movement toward or away from a stimulus, as in the heliotropic growth of plants in the direction of sunlight).
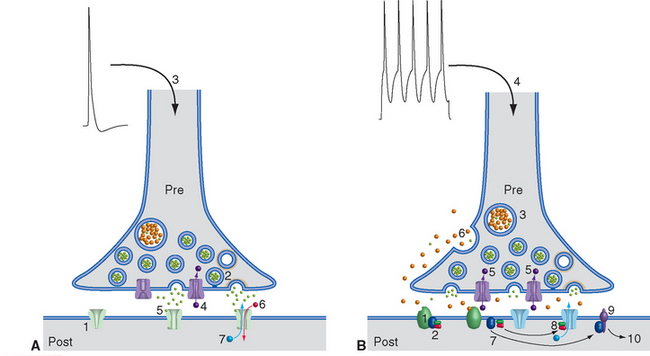
Figure 8-12 Basic events in typical fast (A) and slow (B) synaptic transmission. A, At rest, ligand-gated ion channels (ionotropic receptors) in the postsynaptic membrane are closed (1), and vesicles are docked in the presynaptic terminal (2) awaiting release. Depolarization of the terminal (3) causes Ca2+ influx (4), transmitter exocytosis, binding of transmitter to postsynaptic ligand-gated ion channels (5), and opening of the channels. In this example, open channels allow Na+ influx (6) and K+ efflux (7), depolarizing the postsynaptic membrane. B, Most slow synaptic responses involve metabotropic receptors (1) coupled at rest to a three-subunit G protein (2), as well as transmitters in large, dense-core vesicles (3) or in certain small vesicles. Prolonged or repetitive depolarization (4) of the presynaptic terminal causes widespread Ca2+ influx (5), exocytosis of transmitter from large, dense-core vesicles (6), and binding to G protein–coupled receptors (some of which may be located some distance from the presynaptic terminal; see Fig. 8-9). This binding causes dissociation of the G protein into subunits (7), which can have a variety of effects. They may bind to ion channels and alter their conductance (8); activate an enzyme (9), which in turn alters the concentration of a second messenger (e.g., cyclic adenosine monophosphate, inositol triphosphate, arachidonic acid metabolites); or have even more complex effects (10), such as altering gene expression.
Slow Synaptic Transmission Usually Involves Postsynaptic Receptors Linked to G Proteins
Slow synaptic potentials are also caused by changes in current flow through membrane ion channels, but in this case a multistep process is involved in which binding of neurotransmitter leads to altered concentrations of second messengers, which in turn modulate channel conductance. Because of the intermediate metabolic events, the receptors are referred to as metabotropic receptors. Most metabotropic receptors are membrane-spanning proteins linked to adjacent guanine nucleotide-binding proteins (G proteins). Transmitter binding by a G protein–coupled receptor causes dissociation of subunits of the G protein, which are then able to move laterally in the postsynaptic membrane and exert other effects (Fig. 8-12B).
In the simplest scenario, a G protein subunit directly influences the state of ion channels, for example, causing K+ or Ca2+ channels to open; in this instance, the G protein subunit itself is the second messenger. In most cases, however, the G protein subunit increases or decreases the activity of an enzyme, which in turn causes changes in the concentration of something else.
Multistep pathways such as this are slow and seem unwieldy, but they actually have considerable advantages. Synaptic inputs can be amplified, because a single receptor can cause the dissociation of multiple G proteins, and changing the activity of an enzyme can cause the synthesis or degradation of thousands of second messenger molecules. In addition, because there are many types of G protein–coupled receptors and many types of G proteins, a wide array of postsynaptic effects can result. Some, as indicated previously, are as simple as opening or closing an ion channel. Others may actually be electrically silent, causing things such as changes in sensitivity to other transmitters or changes in gene transcription. This kind of strategy is so versatile that it is not restricted to synapses. G proteins and second messengers are the basis for the receptor potentials generated by retinal rods and cones, olfactory sensory neurons, and some other receptor cells (see Chapter 9).
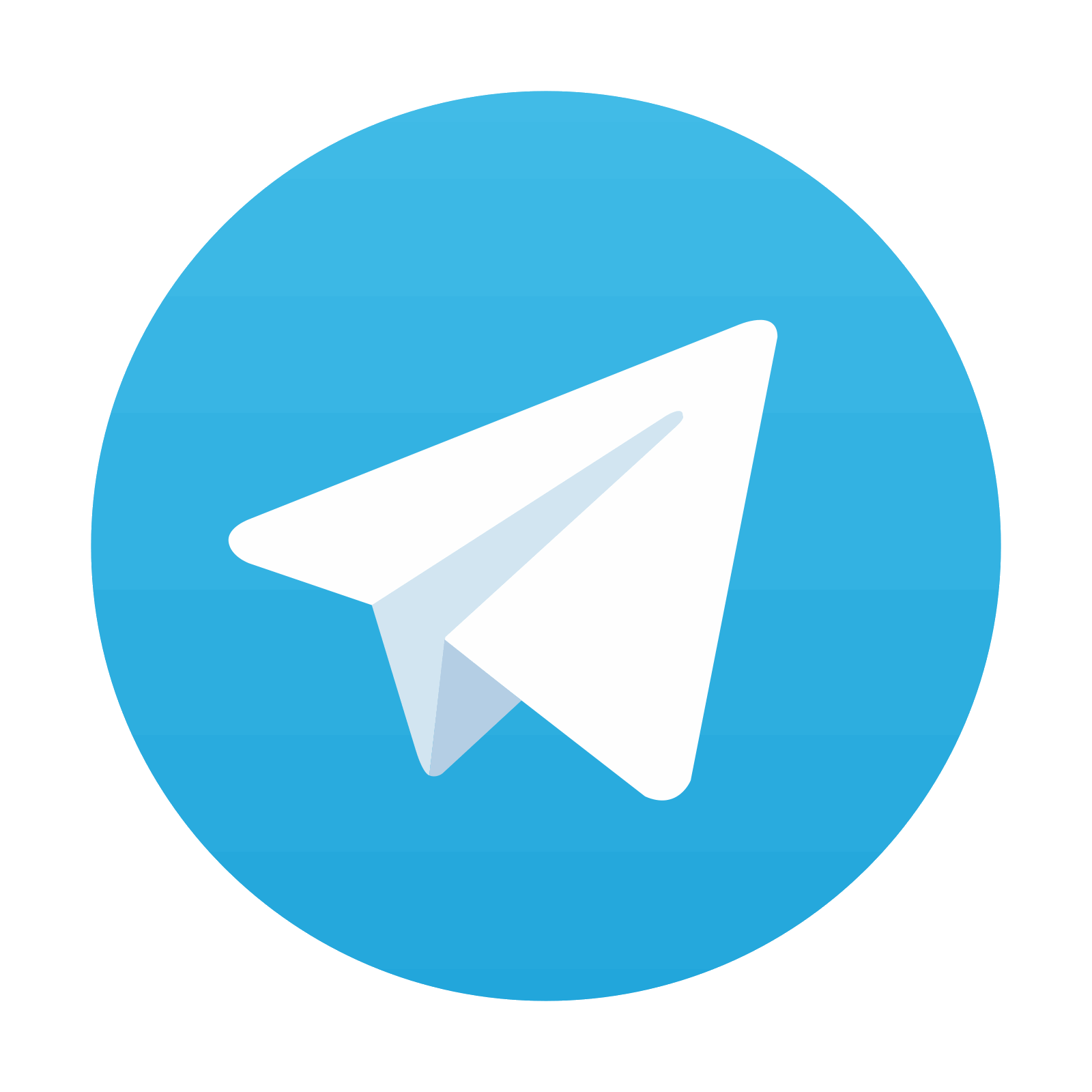
Stay updated, free articles. Join our Telegram channel
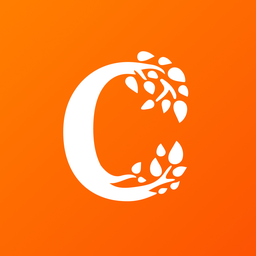
Full access? Get Clinical Tree
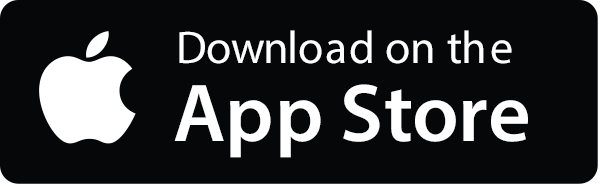
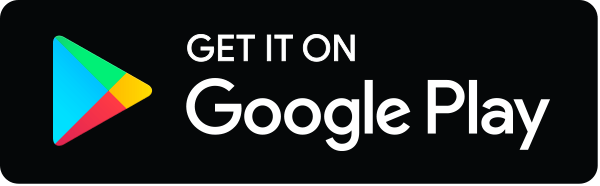