Localization and Field Determination in Electroencephalography and Magnetoencephalography
Richard C. Burgess
Masaki Iwasaki
Dileep Nair
LOCALIZATION AND MAPPING
The word electroencephalogram (EEG) was derived from Greek roots to create a term meaning an electrical picture of the brain. While interpreting an EEG, electroencephalographers maintain a three-dimensional picture of the brain/head in their minds. In principle, there are an infinite number of different source configurations of an electrical event within the head that may give rise to the same electrical and magnetic field distribution at the scalp. Despite this theoretical constraint, one of the key functions of the electroencephalographer is to conceptualize the generators in relationship to this vision and to build an increasingly clear mental image of the foci of these generators. Methods for localization and field determination are tools to help the electroencephalographer infer the location, strength, and orientation of generator sources within the cortex, based on their manifestation at 21 or more EEG recording sites. The same generators that originate and spread their activity for measurement by EEG also produce magnetic fields that can be picked up by extremely sensitive detectors—that is, magnetoencephalography (MEG).
Scalp electrical activity arises from both physiologic and pathologic brain generators. Localization of epileptiform potentials from scalp EEG is critically important for pinpointing the epileptic focus and identifying the region of brain pathology (1). Many electroencephalographers take a simplistic approach, assuming that the generator source must be close to the point where the maximum voltage is recorded. Attempts to systematize the localization of specific EEG activity date back to the early years of electroencephalography. In the mid-1930s, Adrian and Matthews (2), as well as Adrian and Yamagiwa (3), used phase-reversal techniques to localize normal rhythms, and Walter (4) used phase reversals for localization of abnormal EEG activity, as did Gibbs and Gibbs (5) in 1941 in their classic atlas. More recently, a variety of reviews have outlined general principles for the use of polarity, montages, and localization (5, 6, 7, 8, 9, 10, 11). It should be emphasized that phase reversals are not inherently an indicator of abnormality. Phase reversals occur as a result of both normal and abnormal activity. Phase reversals are most obvious in sharply contoured transient activity and, therefore, provide a dramatic visual clue in the case of epileptiform abnormalities.
Despite the critical importance of accuracy in localization, there has been an absence in the literature of descriptions of systematic methods for accomplishing this localization in a simple, manual fashion (12,13). Most textbooks emphasize the distribution that would occur as a result of an assumed generator (i.e., the “forward” problem). With the evolution of digital EEG (14), a variety of methods for computerized source localization have become available (15,16) at the push of a button within the EEG machine itself or as stand-alone software (17,18). These techniques are primarily model-based and have significant limitations; they have generally not been used in routine clinical practice. A practical guide for the step-by-step identification of the origin of epileptiform activity was developed at the
Cleveland Clinic Foundation (19) and is discussed in detail in this chapter. This review concentrates primarily on defining the electrophysiologic origin of epileptiform activity.
Cleveland Clinic Foundation (19) and is discussed in detail in this chapter. This review concentrates primarily on defining the electrophysiologic origin of epileptiform activity.
New imaging techniques have decreased the importance of EEG for many neurologic disorders, but EEG is still the sine qua non for the diagnosis of epilepsy. Although sometimes marketed as an “imaging” tool, MEG senses brain electrical behavior in a way similar to that of conventional scalp EEG (20). With MEG as well, a systematic way to arrive at the sources of the observed magnetic fields is required, quite analogous to that with EEG. Despite the relatively high equipment cost, MEG possesses a number of inherent advantages: (a) It requires simpler forward modeling, resulting in an inherently higher source resolution; (b) recordings are reference-free; (c) signals are not attenuated by bone and scalp; and (d) it is easy to obtain multichannel, whole-head, high spatialdensity recordings. As MEG becomes increasingly available, it may play a more important role in the localization of epileptic discharges.
There are two steps in the interpretation of epileptiform discharges: surface field determination and source localization. Proper determination of the field distribution, based on the manifestations detected by either electrical or magnetic sensors, results from correct interpretation or calculations, and there is only one answer. Accurate field determination is essential not only for accurate source localization, but also for discrimination of epileptic activity from other nonepileptic transients. For source localization, on the other hand, no single unique solution exists. To arrive at a plausible solution for the source location, several assumptions are useful. In this chapter, practical neurophysiologic concepts that relate the generator to the surface electrical fields are described in the first section. The next three sections describe important conventions used in visual interpretation of EEG regarding instrumentation, field determination, and source localization. Last, the principles of MEG recording and its complementary perspective on source localization of the very same signals are reviewed.
PRACTICAL CONCEPTS OF ELECTRICAL FIELDS APPLIED TO BRAIN GENERATORS
Sources
The electrical sources seen at the scalp arise from intracranial focal dipoles or sheets of dipoles. These dipoles represent the postsynaptic potentials originating from vertically oriented neurons. A unit current dipole is created by the intercellular laminar currents in the apical dendrites arising from the pyramidal cells in the outer layer of the cerebral cortex. Specifically, superficial excitatory postsynaptic potentials and deep inhibitory postsynaptic potentials generate almost all spontaneous EEG activity (21), particularly the epileptogenic abnormalities. When populations of neurons are more or less synchronously activated for relatively long durations, the activity can be macroscopically recorded from a certain distance as a linear summation of the unit dipoles (22, 23, 24). This summated activity may also be represented as a dipole or sheet of dipoles along the cortex. Thus, the generator of epileptic activity can be explained by a single or multiple equivalent current dipoles (8,13,25,26). The surface potential can be thought of as a two-dimensional projection, or shadow, of a complex three-dimensional electrical object residing inside the head.
Fundamental to scalp localization is the concept of the “inverse” problem, which entails an estimate, based on surface data, of the magnitude, location, and distribution of electrical fields throughout the brain. Whereas the forward problem is solvable with unique solutions, the inverse problem is not. Nunez (9) described the mathematical representation of the biophysics underlying volume conduction.
The forward problem can be stated as follows: Given known charge distributions and volume conductor geometries and properties, predict the resulting surface potential distribution. The solution involves applying numerical or analytical methods for any known set of geometries and boundary conditions (9,27) to solve Poisson’s equation:
In this equation,
2 is the second spatial gradient operator, Φ is the scalar potential in volts, ρ is the free charge density, and ε is the permittivity of the mass of tissue. Multiple sources can be shown to combine linearly, so that a combination of sources results in the arithmetic sum of the potential distributions that each would produce individually.

The corresponding inverse problem can be stated as follows: Given a surface potential distribution and the volume conductor geometries and properties, determine the underlying charge distribution. Unfortunately, a given surface map can be produced by any of an infinite number of possible source distributions. EEG and MEG record at a distance from the sources, and they use only a limited number of sensors—typically, 20 to 100 for scalp EEG and 100 to 300 for whole-head MEG. Therefore, this problem generally has no unique solution (28). Nevertheless, simplifying assumptions are possible.
The single-dipole solution is the most frequently sought interpretation of scalp potential distributions and is the solution produced by most “dipole localization” systems in current use. Such a solution should account for a maximum amount of the observed map, but a residual “error” term may remain. In addition, the following assumptions are made: (a) the source dipole is near the surface; (b) the source dipole is perpendicular to the surface; (c) the head is a uniform, homogeneous volume conductor; (d) at least
one recording electrode is essentially over the source; and (e) the reference is not contained in the active region.
one recording electrode is essentially over the source; and (e) the reference is not contained in the active region.
On the basis of these assumptions, one generally looks for a single predominating potential maximum on the surface, with the source lying directly below it; however, a variety of nondipolar source configurations could produce the same observation. For example, a simple monopolar charge buildup, a curved dipole sheet, or a finite-thickness dipole “pancake” would produce a single well-defined maximum. In addition, signals originating from confined, but deep-seated, generators are broadly distributed when recorded from the surface (29,30), and cannot be reliably distinguished from more superficial, but widespread, epileptic regions.
In addition to these “equivalent” source possibilities, others are physiologically similar but generate very different surface maps. Through variations in orientation and shape, dipole sheets can produce charge reorientation and cancellation. The resultant range of possible scalp distributions serves as a reminder that observed scalp maxima do not necessarily lie directly above maximal brain activity. Jayakar and colleagues (31) pointed out the difficulties involved in localizing epileptic foci on the basis of simple models, owing to effects from dipolar orientation, anatomic variations, and inhomogeneities, among other factors.
Volume Conduction
In a volume conductor, the electrical field spreads instantaneously over an infinite number of pathways between the positive and negative ends of the dipole. Outside the neuron, the circuit is completed by the current flowing through the extracellular fluid in a direction opposite to the intracellular current. Through the process of volume conduction, electrical activity originates from a generator and spreads through a conductive medium to be picked up by a distant recording electrode. Volume conduction is passive—that is, it does not involve active regeneration of the signal by intervening neurons or synaptic relays—and occurs as easily through saline as it does through brain parenchyma. Potentials recorded by way of volume conduction are picked up synchronously and at the speed of light at all recording electrodes. Although attenuated with distance by the medium, volume-conducted components preserve their original polarity and morphology.
The attenuation factor is defined by the inverse square law—that is, the recorded electrical potential falls off in direct proportion to the square of the distance from the generator (32,33). For example, a 100-mV potential seen at the electrode directly overlying a cortical generator (assume a distance of 1 cm away) will be reflected as a 4-mV potential at an electrode that is 5 cm away and as only a 1-mV potential at 10 cm away. The rapidity of this falloff is a function of the depth of the generator, with more superficial generators falling off much more rapidly. Distant generators have a “flat” falloff, one of the hallmarks of a “far-field” potential (Fig. 11.1).
The medium through which current travels to reach the recording electrode is not homogeneous, but rather exhibits a variety of conductivities (34). As the current attempts to complete its circuit by following the path of least resistance, these differences in conductivities, especially differences among cerebrospinal fluid (CSF), skull, and scalp, and their associated boundaries, affect the electrical potential recorded on the scalp. The signal is not only altered in amplitude, but also low-pass filtered during its passage to the surface because of the capacitive and shunting effects of the intervening layers. In the skull, the
conductivity in a tangential direction is higher than that in a direction perpendicular to the surface. This produces a “smearing effect” on the surface potential distribution (35). Although the current tends to flow along the path of least resistance, there is still some flow throughout the volume conductor, thereby permitting recording of the electrical potential at all sites on the volume conductor, albeit with the amplitude inversely related to the square of the distance from the source (Fig. 11.1).
conductivity in a tangential direction is higher than that in a direction perpendicular to the surface. This produces a “smearing effect” on the surface potential distribution (35). Although the current tends to flow along the path of least resistance, there is still some flow throughout the volume conductor, thereby permitting recording of the electrical potential at all sites on the volume conductor, albeit with the amplitude inversely related to the square of the distance from the source (Fig. 11.1).
The head also contains normal or abnormal openings that present low-resistance paths to conducted currents. The current tends to flow toward skull defects, whether physiologic (such as foramina) or acquired through trauma or surgery, and around cavities (such as the ventricles), markedly distorting the field in the region of the defect. The resistivity of scalp or brain tissue is many times smaller than that of bone (36, 37, 38). As a result, surface potentials near these openings will be unusually high, and the largest potentials can be seen at the location of the defect even when the source is several centimeters away from the defect (35,39,40). MEG signals are less distorted by these heterogeneities in conductivity.
Surface Electrical Manifestations
A variety of real-world considerations complicate the interpretation of surface recordings. Because the dipoles measured at the scalp are ordinarily oriented radially, scalp electrodes “see” primarily the positive or the negative pole. Although generators located at the apex of a gyrus lie perpendicular to the scalp (i.e., vertical dipoles), any generator within a cortical fissure will present a dipole at an angle to the scalp. Nearly 70% of the cortical surface lies within the sulcal depths (41). In addition, many brain areas—most notably, the mesial frontal, parietal, occipital, and basal temporal cortex—are diversely oriented and lie at varying distances from surface electrodes. Hence, it is not sufficient to assume that the generator must be close to the point where the maximum potential is recorded (7). Finally, the choice of reference affects the form of the EEG measurements.
When a generator dipole is oblique or parallel to the scalp, the resulting surface potentials can lead to false localization of the potential maximum. The typical bellshaped distribution of the electrical field is replaced by one shaped like a sideways “S.” Because both the positive and the negative ends of the dipole may be recorded at the scalp, the surface potential can exhibit two “maxima” of opposite polarity. Between the two ends is a zero isopotential boundary where the generator will not be picked up at all.
It is important to distinguish true horizontal dipoles, such as those arising at a sulcus or the interhemispheric fissure, from field distributions resulting from widely separated activity but giving rise to distinct negative and positive maxima. For example, bisynchronous temporal spikes differing slightly in phase, such that the negative component on the left aligns with the positive component on the right, may appear to represent huge transverse dipoles (31); however, careful evaluation with an alternative reference (or the demonstration that the spikes also occur asynchronously) can prove that the fields represent not the source and sink of a single dipole, but rather two generators (42) linked by corticocortical propagation.
When a source lies deeper in the brain, two changes occur: the surface potential becomes smaller, and the field becomes more widespread relative to the surface maximum (29,30,42). Although the shape of the electrical field gradient can indicate the type of field and the distance of the generator, identifying the source on the basis of the potential difference between any scalp electrodes becomes increasingly more difficult. When the potential field gradient is relatively flat, as is the case in the far-field potential from a deep-seated source, a bipolar montage will display the waveform at relatively smaller amplitude (Fig. 11.1). Diffuse discharges may be better appreciated on referential montages, assuming that the reference is not involved. An adequate “vantage point” may be impossible with surface electrodes when a focus is deep. It may be impossible to find a scalp electrode reference that is not electrically involved in the active region, and some cases can only be resolved by invasive electrode placements that can monitor more limited areas (30,44, 45, 46, 47).
The combination of multiple sources can produce a variety of results. A superficial source can overshadow a deep one, distorting or even hiding it. Because the amplitude of a measured potential is inversely proportional to the square of the distance from the recording electrode, nearby sources can appear disproportionally higher at the recording electrodes. A given electrode thus has a “view” of the nearby generators, such that dipoles that combine to reinforce each other will have a large net effect, whereas those that cancel will produce a smaller or null potential (48).
Complicating this problem is the fact that the equivalent dipole is an abstraction. In reality, only sources that extend over multiple layers of several square centimeters of cortical tissue have sufficient energy to generate detectable scalp discharges (43,49). An epileptogenic zone almost always consists of a continuum of dipoles, resulting in a sheet, or “patch” (50), dipole. Such a source may cover an extended brain region, with the constituent areas lying at various depths and orientations. Again, it is possible for both reinforcement and cancellation to produce a variety of surface potential distributions. Overall, the conduction phenomena leading to surface potentials follow the “solid-angle” rule (51)—that is, the net surface potential is proportional to the solid angle subtended by the recording electrode. Unless a dipole sheet parallels the surface, the maximum surface potential may be somewhere other than directly over the affected area, as illustrated in Figure 11.2. The solid-angle theorem helps to explain the results of multiple synchronously discharging pyramidal neurons arrayed over a cortical region containing both sulci and gyri.
In the same way that opposing dipoles can cancel each other relative to a distant electrode, a sheet of nonparallel dipoles can produce a “closed” field (52) whose potential contributions will cancel, resulting in a negligible potential at the surface (53). These generators, usually not visible on scalp EEG, are observed primarily on invasive recordings (48). Even when not a completely closed field, multipolar source-sink configurations tend both to produce more cancellation than dipolar generators and to attenuate more quickly as a function of distance (9). This irregular structure is particularly likely in the basal and mesial areas of the temporal cortex and the hippocampus, where cortical infolding is so prevalent (54).
The head consists of a series of roughly concentric layers that separate the brain from the scalp surface. Each of these layers—CSF, meninges, bone, and skin—presents different electrical characteristics to the currents that conduct the EEG to the surface. These layers occasion considerable current spreading, which causes the potential for localized foci to appear in a much broader scalp area (9,55). Spreading in itself is not an insurmountable problem, because it is theoretically possible to recover deep dipole sources based on observed surface potentials, using appropriate mathematical transformations. Such recovery, however, is guaranteed only in a perfectly spherical concentric conductor, onto which electrodes can be placed in any location. The head is not a perfect globe, however, and significant constraints disqualify the face or neck, which may be preferred for certain sources, as electrode sites.
Electrode Placement as Spatial Sampling
Placement of scalp electrodes should be considered an exercise in spatial sampling. Electrode density must be generous enough to capture the available information but not so closely spaced as to overwhelm with redundant data. Inability to precisely locate a cortical generator may be the result of spatial undersampling (i.e., “aliasing”). The assumption that a potential will decrease monotonically as distance increases from the involved electrode is based not only on an uncomplicated electrical field—that is, a monopole—but also on an electrode placement sufficiently dense to accurately represent the spatial contours of the field. Cooper and associates (49) suggested that 6 cm2 or more of cortex discharging simultaneously is required to reflect a visible potential on the scalp surface. Because most epileptogenic potentials seen on the scalp are visible at multiple electrodes, a considerably larger cortical area must be synchronously discharging to produce these potentials. The widely accepted International 10-20 Electrode Placement System (56), although relatively easy to apply reproducibly, has some inherent limitations in terms of the accuracy of localization (57). When more precise localization is indicated to avoid spatial aliasing, scalp electrodes should be placed at least once every 2.5 cm (58). The maximum spacing can be determined theoretically (59) as well as experimentally, and as many as 128 electrodes (spaced approximately 2 cm apart) is sometimes necessary (60).
Boundary Problems
Regardless of the fineness of the scalp electrode grid, boundary effects will occur at the edges of the array. The maximum potential must be well within the scope of the recording electrodes to ascertain that a physiologic gradient exists away from the electrode. For example, epileptic sharp waves arising from mesial temporal structures are frequently localized outside the area covered by the 10-20 Electrode Placement System (61, 62, 63, 64). It is impossible to determine the complete extent of the maximum fields unless the area is surrounded by regions of lesser activity. Recordings in which the activity is large all the way to the boundary of the region defined by the montage must be “remontaged” to include, if possible, all the relevant electrodes, or further recording must be done with additional electrodes. This may be especially complicated when it is difficult to position electrodes inferior to the customary borders of scalp coverage.
A significant portion of the head cannot be practically surveyed, and important brain areas, such as the basomesial temporal cortex and other deep sources, are only indirectly accessible with standard scalp electrodes. Additional electrodes inferior to the 10-20 Electrode Placement System (56) must be used to provide a better view. In certain circumstances, the information obtained from a combination of closely spaced scalp electrodes, such as the International 10-10 Electrode Placement System (65, 66, 67), and sphenoidal electrodes can obviate the need for more invasive recordings (68).
ELECTROENCEPHALOGRAM INSTRUMENTATION CONSIDERATIONS RELATED TO LOCALIZATION
Differential Amplifiers
Amplifiers used in clinical neurophysiology measure the difference between two potentials at the inputs to the amplifier and provide an amplified version of this difference at the output. These devices, called differential amplifiers, eliminate unwanted signals that are identical at both inputs, called common mode signals. The two terminals at the input to a differential amplifier are sometimes labeled G1 and G2, recalling when a grid within the vacuum tube amplifier controlled the flow of electrons from cathode to plate. Modern operational amplifier (op-amp)-based differential amplifiers use complex integrated circuits, and the terms “input 1” and “input 2” are used throughout this chapter.
The amplifier itself has no concept of polarity; it simply does the subtraction and the gain multiplication, and then provides an output voltage that is a linear function of the input voltages, according to the following equation:
Voutput (t) = G × [Vinput1 (t) − Vinput2 (t)]
In this equation, Voutput (t) and VinputN (t) are the output and input voltages, respectively, and G is the gain of the amplifier. It is only during interpretation of the EEG waveform in the context of the underlying generators that the concept of polarity has any meaning. Inexperienced electroencephalographers often mistakenly ascribe a polarity at the input to a specific pen deviation at the output (12). It should be remembered that there are no positive deflections and no negative deflections; there are only upward and downward deflections (12). Figure 11.3 illustrates four different input conditions that give rise to exactly the same deflection.
Polarity Conventions
Deflection refers to the direction on the page or display screen in which the waveform component under study appears to go, and it is a function only of the display instrumentation. By EEG convention, upward deflections are caused by input 1 being more negative than input 2. Downward deflections are caused by input 1 being more positive than input 2. These relationships imply nothing about the underlying polarity of the signals at inputs 1 and 2, only about the polarity of their differences. When the name of the electrode connected to input 1 is written above the deflection and the name of input 2 below, the deflection will point to the electrode with the “relative” negativity, as in Figure 11.4.
If the difference between the two signals at the input is zero, no deflection will occur. When two electrodes (no matter how close to the source of the sharp wave or spike) that lie along the same isopotential line (typically at the same distance from the generator) are input to a differential amplifier, the output will reflect no activity, even though both electrodes may be measuring high amplitudes in an absolute sense. Some amplifiers used in basic neurophysiology research and in clinical evoked potentials use another convention, designed to display positive input 1 as an upward deflection.
Derivations and Montages
A derivation describes the connections of the electrodes to the amplifier inputs. A montage is a combination of
derivations arranged down the EEG page to display many amplifier channels simultaneously in a way that aids in the identification and localization of abnormalities (69). Each amplifier could be connected to any pair of available electrodes. Likewise, these amplifier outputs could be arranged in any fashion on the screen; the arrangement in chains assists our visual localization capabilities.
derivations arranged down the EEG page to display many amplifier channels simultaneously in a way that aids in the identification and localization of abnormalities (69). Each amplifier could be connected to any pair of available electrodes. Likewise, these amplifier outputs could be arranged in any fashion on the screen; the arrangement in chains assists our visual localization capabilities.
The arrangement of derivations into a montage determines whether it is called bipolar or referential. Derivations in bipolar montages are established between neighboring electrodes to emphasize focal activity. They take advantage of the subtractive nature of differential amplifiers to effect a high degree of cancellation. Any montage can be analyzed to locate the maximum of a sharp wave or spike, provided that the montage has a logical order (6,70,71).
ELECTRICAL FIELD DETERMINATION ON THE SCALP
Identification of Peaks and Measurement of the Amplitude
Interictal epileptiform abnormalities are recognized by their morphology—an impression of “standing out” from the background—and by their electrical field distribution, which must demonstrate a realistic relationship between the electrical potentials at topographically associated electrode positions. In choosing an abnormality to localize, the peak selected must be representative of the patient’s population of spikes, and the sample must be as clean as possible.
It is assumed that an activity starts from zero and reaches its maximum after a certain time. The amplitude of the activity is measured between the zero and the maximum peak. However, it is often difficult to identify the level of the “zero” in each EEG channel correctly, because the activity is superimposed on the background, arises from the noise level, or continues from the preceding activity. Sometimes sharp activity can be separated from a slower background, provided the frequency of the epileptic activity is clearly different, by using filtering.
Practically, the amplitude is measured as peak-to-peak or baseline-to-peak. Identification of the baseline and peak may be particularly troublesome in the case of polyphasic discharges, in which each phase is brief and difficult to line up temporally. When analyzing a peak, the maximum value in each EEG channel should be identified at exactly the same time point. During visual analysis of a waveform, the montage selected will influence identification of the peak, resulting in different, or sometimes erroneous, field determinations. Multiple peaks or phase reversals with small time shifts reflect a sequential change in the location of the maximum. In the EEG tracings shown in Figure 11.5, note that the peaks of several of the channels were reached on different phases of the waveform, giving the erroneous appearance of a phase reversal. Computerized source localization techniques are especially sensitive to the selection of the appropriate time frame. Errors in identifying the peaks that are to be mapped can cause extraordinary displacements in the apparent localization of the sources (72,73).
The peak of the sharp wave (i.e., the negative extrema) generally has the highest amplitude at the electrode closest to the involved cortical epileptogenic neurons (7). The main component of an epileptic discharge may be preceded by a smaller deflection of the opposite polarity. Early components show a more localized field than do later ones (74,75), and they are more synchronous than the slow wave that frequently follows a spike. Thus, the initial deflection probably contains more localizing information (31), and using the lower-frequency waves for localization may not always represent the epileptogenic region.
Mapping the Electrical Field
Two-dimensional display of the scalp regions involved in epileptiform or other activity is called mapping. Isopotential lines are drawn on a representation of the scalp to specify the topography of equivalent electrical potentials, similar to the isocontour lines drawn by a surveyor on a land map.
The potential fields of spikes and sharp waves can be mapped even without electronic assistance by tracking the relationships of the electrical potential level between electrodes. As the initial step, a longitudinal or transverse chain of the electrodes is used to map the one-dimensional relationship of voltage level to electrode position, as illustrated in Figure 11.6 (top). Then two chains are connected to each other through a common electrode to obtain the
two-dimensional relationship. To create an isopotential contour map, a 100% value is assigned to the maximum and a 0% value to the minimum. However, as discussed (in source localization choosing between two possibilities) the polarity of the maximum depends on an assumption about the generator. The maximum may be the highest negative point or the highest positive point. Similarly, the minimum may be negative or positive, or may have a mid-curve value when two maxima of opposite polarities are assumed—for example, a horizontal dipole generator. Depending on the polarity of the maximum—that is, the point given a 100% value—at least two different isocontour maps can be obtained, as shown in Figure 11.6. To make the correct choice, some assumptions must be introduced, as is described in source localization assumptions.
two-dimensional relationship. To create an isopotential contour map, a 100% value is assigned to the maximum and a 0% value to the minimum. However, as discussed (in source localization choosing between two possibilities) the polarity of the maximum depends on an assumption about the generator. The maximum may be the highest negative point or the highest positive point. Similarly, the minimum may be negative or positive, or may have a mid-curve value when two maxima of opposite polarities are assumed—for example, a horizontal dipole generator. Depending on the polarity of the maximum—that is, the point given a 100% value—at least two different isocontour maps can be obtained, as shown in Figure 11.6. To make the correct choice, some assumptions must be introduced, as is described in source localization assumptions.
The ideal situation in referential recording occurs when the reference electrode is totally inactive or picks up activity of negligible amplitude. In this situation, those channels showing some activity will deflect in one direction only, as illustrated in Figure 11.7 (bottom). The electrode closest to the generator will show the largest deflection, with the amplitude of the deflection in all the other channels directly proportional to the magnitude of activity recorded from each of those electrodes. This situation makes it especially easy to find the maximum and to assess the extent of the field distribution (Fig. 11.7). To achieve this ideal situation, an alert technologist will recognize a contaminated reference and construct a “distribution montage,” typically with a reference electrode from the other hemisphere (Fig. 11.8).
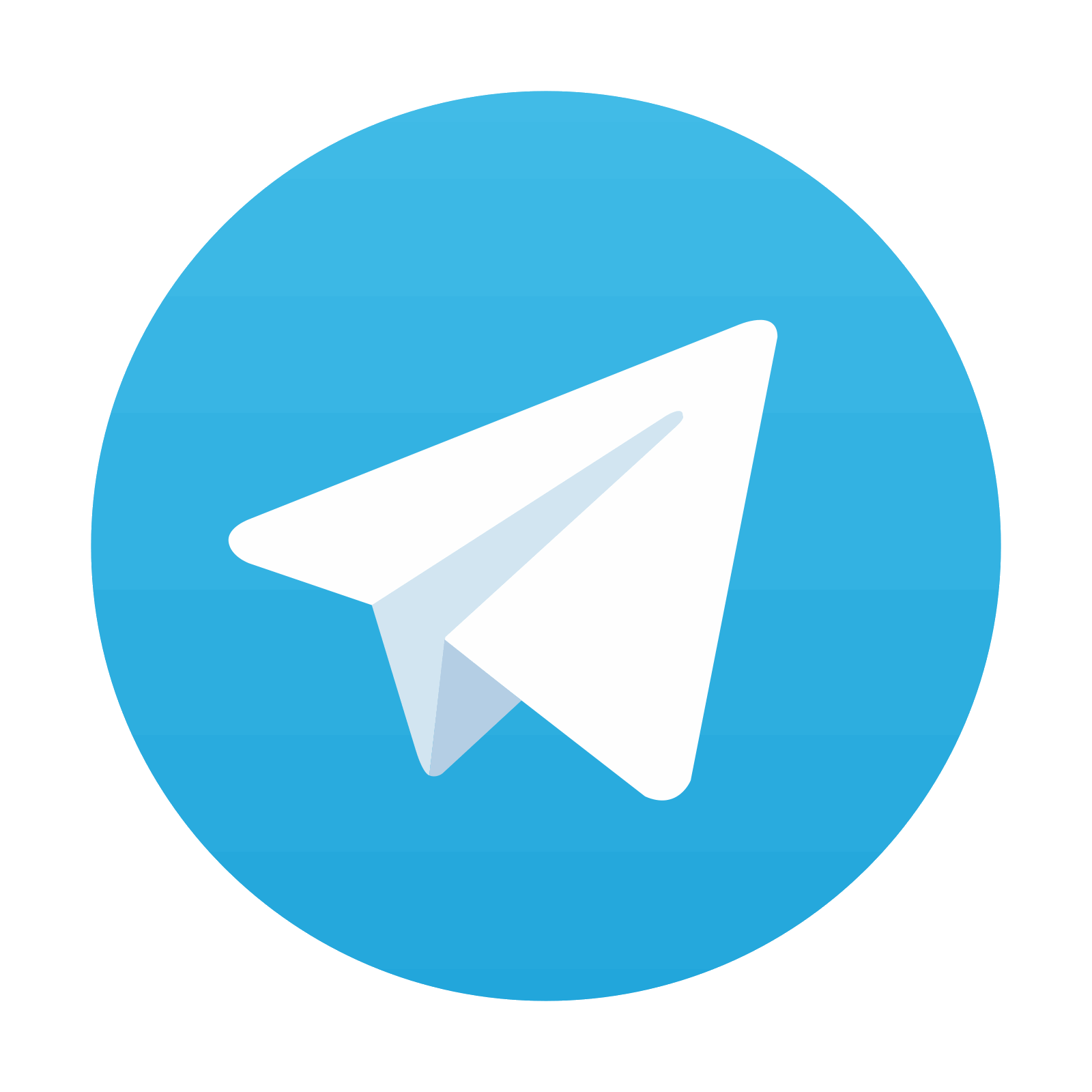
Stay updated, free articles. Join our Telegram channel
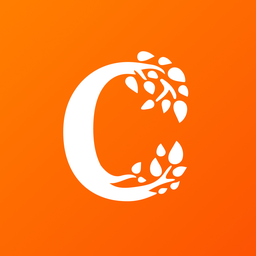
Full access? Get Clinical Tree
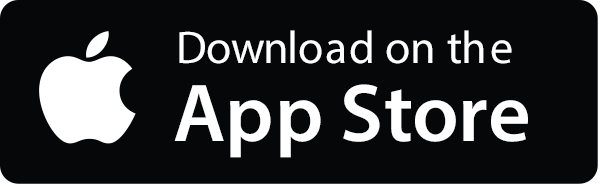
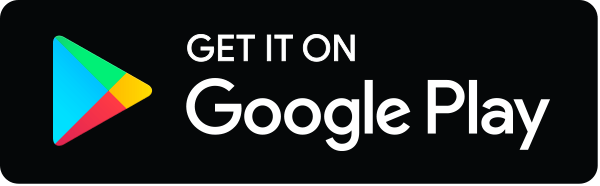