Introduction
The basic tenet of neurocritical care is prevention of secondary injury. Secondary injuries following the initial ictus include global increases in intracranial pressure (ICP), alterations in cerebral perfusion, hydrocephalus, and seizures, among others. In addition, systemic insults such as infections, hypotension, thromboemboli, hyperglycemia, hyperthermia, malnutrition, and other complications can affect patients in the neurocritical care unit (NCCU) adversely. Accumulating evidence suggests that inflammation can exacerbate secondary injury in brain disease and in particular subarachnoid hemorrhage, vasospasm, traumatic brain injury (TBI), and stroke; it can even be epileptogenic. Monitoring neuroinflammation therefore may allow better understanding of the impact of therapies aimed at preventing or minimizing secondary injury. Whether prevention of inflammation will improve patient outcome following brain injury is still to be defined because although pharmacologic therapies to attenuate inflammation can ameliorate pathology, microglial activation that is central to the inflammatory response also plays a role in recovery and regeneration (i.e., inflammation may have a dual role).
Monitoring Neuroinflammation
As the understanding of the pathophysiologic process following acute brain injury including subarachnoid hemorrhage (SAH), stroke, and TBI has increased, awareness about the deleterious effects of inflammatory activation as both a trigger and exacerbating factor of secondary injury has created new opportunities in monitoring neuroinflammation. For example, findings from clinical studies suggest that neutrophils play a critical role in ischemia-reperfusion injury, because tissue damage is associated with extent of neutrophil infiltration. The critical role that systemic as well as local inflammatory activators play in determining the extent of central nervous system (CNS) injury following an ictus is highlighted by the emergence and widespread use of anti-inflammatory pharmaceuticals, such as statins, in neurocritical care. 3-Hydroxy-3-methylglutaryl coenzyme A (HMG CoA) reductase inhibitors, commonly known as statins, have lipid-lowering properties, and animal models of acute brain injury have demonstrated their anti-inflammatory properties. There is mounting evidence for the role of neuroinflammation as a contributor to secondary brain tissue injury in stroke, neurotrauma, SAH, and various chronic CNS diseases. Inflammatory mediators are indeed elevated in the cerebrospinal fluid (CSF) and serum of patients with acute and chronic brain injury, and are associated with poor outcome. Various animal models also point to potential protective mechanisms with the inhibition of proinflammatory mediators. As the role of neuroinflammation in secondary brain injury becomes more evident, the need to monitor its activity in the NCCU becomes important.
The knowledge that may be gained by monitoring neuroinflammation and its clinical utility can be organized into three main areas:
- 1.
Identifying risk factors to predict secondary injury
- 2.
Providing endpoints to monitor responses to specific therapies aimed at modulating the inflammatory state
- 3.
Gaining insights into the pathophysiology of the disease process
Monitoring of neuroinflammation thus may provide a more sensitive and earlier detection of secondary injury and in turn widen the therapeutic window. Furthermore, studying the response of the various measured parameters to conventional and experimental medical and interventional therapies should augment our knowledge of the disease course and potentially lead to new therapeutic strategies.
Basic Science of Inflammation
The brain was once considered to be an “immune-privileged” organ system, inaccessible and independent from systemic inflammation. Although the CNS has unique features, this view has largely fallen out of favor because there is accumulating evidence of crosstalk between the CNS and the immune system outside of the CNS. For example, CNS cells have been shown to modulate peripheral immune function in response to peripheral disease and injury through the systemic release of various cytokines (i.e., interleukin-1 [IL-1], IL-6, tumor necrosis factor-α [TNFα]) and vagal efferents.
Cerebral edema has many potential causes but can be considered an inflammatory process. Indeed IL-1 neutralizing antibodies in animal models can attenuate brain edema. Because the skull is functionally a closed box, significant development of edema occupies the excess space in the cranium, after which pressure increases and so can damage neurons and glia. Cerebral edema occurs at different times in different cerebral injuries. In TBI and SAH, cerebral edema develops quickly and can cause increased ICP in many patients. Conversely, in ischemic stroke, cerebral edema develops slowly over the first 72 hours after the initial ictus. The reason for the differences in these time courses is not well defined; however, the course of cerebral edema could be predicted by monitoring inflammation.
Inflammatory processes contribute to CNS injury and neurodegeneration. In addition there is accumulating evidence that inflammation and in particular leukocyte-endothelial cell interactions, play a critical role in the pathogenesis of vasospasm after SAH and that inflammatory processes contribute to formation of cerebral aneurysms. Various types of acute and chronic brain injuries result in gliosis, a reaction characterized by the activation, proliferation, and hypertrophy of mononuclear phagocytic-type cells, which occurs in a well-defined manner to promote a systematic restoration of the brain parenchyma in the setting of neuronal injury. This process is mediated by the resident astrocytes and microglia, which produce various chemical mediators to recruit peripherally derived immune cells to further enhance the inflammatory response. The inhibition of these chemical mediators (i.e., TNFα, IL-1) and the key components that modulate leukocyte trafficking across the blood-brain barrier (BBB) have shown great potential to improve outcome in neuroinflammatory diseases such as multiple sclerosis and hold the same potential in acute brain injury (natalizumab therapy).
Biology of the Brain’s Immune System
Inflammatory processes within the CNS are different from the periphery because the CNS parenchyma lacks resident dendritic cells, with inflammation instead being initiated by the resident microglia. The exact role of microglia remains under debate because they release a number of factors that modulate both secondary injury and recovery after injury, including pro- and anti-inflammatory cytokines, chemokines, nitric oxide, prostaglandins, growth factors, and superoxide species. The most unique quality, however, is the presence of a BBB that regulates the subsequent immune amplification by the peripheral inflammatory cells. The BBB, along with the neuroepithelial cells, modulate the inflammatory mediators and ultimately the peripheral leukocytes that participate in a neuroinflammatory state. These key mediators, namely the cytokines and adhesion molecules, are the focus of current drug development.
CNS injury can result in acute or chronic neurodegeneration. In the acute setting, conditions such as TBI and cerebral ischemia lead to parenchymal injury that is characterized by substantial nerve cell loss from excitotoxicity (i.e., glutamate release), oxidative stress, and regional electrolyte disturbances among other pathophysiologic processes. Proinflammatory cytokines are elevated in human CSF and serum after TBI. In addition, increased microglial activation can be detected in vivo using the positron emission tomography (PET) ligand [11C](R)PK11195 even years after an acute injury. Animal models of acute injury have further implicated these proinflammatory cytokines as contributors to the extent of brain injury because their inhibition can reduce the extent of cell death. Extensive investigations into various conditions of chronic neurodegeneration such as multiple sclerosis and Alzheimer’s disease have also implicated a role for inflammation, because cytokines such as TNFα and interferon-γ (IFNγ) are elevated in the CSF. In addition, in vitro studies point to inflammation’s damaging effects on neurons, whereas cytokine inhibition in animal models slows the disease process. Interestingly, immune responses also are necessary for neuroregeneration, suggesting there is an innate balance between adaptive and deleterious immune activation or immunomodulation.
The entry of leukocytes into the CNS parenchyma depends on the expression of specific molecules by the endothelium (intracellular adhesion molecules [ICAMs], integrins, selectins, and chemokines) along the capillary and postcapillary venules in the brain. The production of chemokines and the activation of the endothelium are initiated by the activated parenchymal microglia, which produce the cytokines and other inflammatory mediators to further stimulate the inflammatory cascade.
The activated endothelium is marked by an up-regulation of cell-adhesion molecules (i.e., selectins and integrins) that act to increase interactions to anchor peripheral leukocytes to promote transmigration. In this multistep paradigm of leukocyte-endothelial interactions to cross the BBB, the first step is tethering, which is characterized by a transient contact between the glycosylated ligands on leukocytes and selectins on the endothelium. The next step is rolling, which is mediated by the shear forces of flowing blood to promote locomotion of the leukocyte and increase exposure to activating factors (i.e., cytokines, chemokines) immobilized on the endothelial luminal surface. Further activation of the leukocytes is characterized by conformational changes of integrins into a higher affinity state for stronger interactions with endothelial cell-adhesion molecules (ICAM-1, vascular cell adhesion molecule 1 [VCAM-1]), resulting in leukocyte arrest onto the endothelial surface. The arrested leukocytes then can extend protrusions enriched in chemokine receptors, and extravasate across the BBB by either a paracellular or transcellular route. Various matrix metalloproteinases (MMPs) are secreted to facilitate the transmigration process.
The Febrile State: Monitoring Brain and Body Temperature
Fever is the oldest and most easily measured marker of an underlying inflammatory condition, with careful records of elevated temperature and its relationship to disease course dating back to antiquity. Raised core body temperature is associated with worse outcome following a variety of acute neurologic insults. The exact mechanism by which hyperthermia exacerbates CNS injury is not yet fully elucidated. Animal infarct models demonstrate that hyperthermia can enhance the depth and extent of injury by one of two mechanisms: increased cytokine release and inflammation or increased energy expenditure and a widened metabolic gap. However, hyperthermia in a brain-injured patient has many disparate causes. First, pharmaceutical and infectious etiologies are common. Second, there may be direct hypothalamic injury. Third, factors such as heat shock protein (HSP) synthesis, release of proinflammatory cytokines such as IL-1, IL-6, TNFα, and interferon, or increased glutamate each, or in combination, may act as the stimulus to trigger fever after TBI (for review see ). Factors that may aggravate neurologic outcome in the setting of hyperthermia include increased glucocorticoid production, increased metabolic expenditure, altered oxygen consumption, glutamate or nitric oxide release, increased myeloid cell and cytokine activity, or increased vascular permeability. Given the complex interplay between factors for fever following SAH, it is not surprising that fever has been found to be an independent risk factor for the development of vasospasm following SAH in some studies, whereas others have not found this relationship. Fever at admission also is a risk factor for vasospasm after TBI and is associated with poor clinical grade after aneurysm rupture.
It is now possible to routinely monitor brain temperature (BT; see Chapter 37 ). Although brain and body temperature may correlate after SAH and TBI, average BT is usually greater than the average rectal temperature by about 1° C. The difference between brain and body temperature becomes greater as a patient becomes febrile. The effect of BT on brain physiology is not well understood. Some studies have demonstrated that fever may be associated with increased ICP, while others suggest that brain hyperthermia may not affect brain oxygen or may even increase it. In part these different results may be associated with changes in cerebral blood volume or cerebral blood flow. On the other hand, microdialysis studies suggest that fever control can attenuate markers of cerebral energy dysfunction in the brain. However, exactly what the relationship between BT and energy dysfunction is, is not clear. The clinical utility of BT monitoring, and in particular the relationship of BT with cerebral metabolism requires more study.
Serum Inflammatory Markers
Systemic indices of inflammation revolve around markers of acute phase reactants such as serum white blood cell (WBC) count and C reactive protein (CRP). Elevation in WBCs can occur in the setting of infection, inflammation, and stress or tissue necrosis. The limitation of these parameters is their nonspecific nature in CNS injury. This can limit an interpretation about CNS inflammation because elevation of these parameters may reflect other organ injury or secondary infection. Despite these limitations, several converging lines of evidence suggest that monitoring acute phase reactants may help develop models to predict secondary injury following brain injury.
SAH is a disease in which inflammation monitoring may be the most advantageous and in particular to evaluate vasospasm. For example, leukocytosis has been found to be an independent risk factor for delayed cerebral ischemia (DCI) following aneurysmal SAH, with each increase of 1000 in the serum WBC associated with a 9% increase in the likelihood of developing DCI. Furthermore, a peak serum WBC greater than 15,000 is associated with a 3.3-fold greater incidence of DCI, whereas a CSF neutrophil content of greater than 62% on the third day after SAH is an independent factor associated with later vasospasm.
The relationship between CRP, vasospasm and the development of DCI is still to be fully elucidated. For example, Rothoerl et al. observed that CRP values were higher in patients who developed DCI 5 to 8 days after aneurysm rupture. This likely represents the response to ischemia. There was a trend observed for higher CRP values on the preceding days but these did not achieve significance. Serum CRP also was greater in poor-grade patients, which makes it difficult to conclude a “causal” relationship between a heightened acute phase response and vasospasm, or that CRP simply reflects the severity of the initial brain injury.
In ischemic stroke, CRP and leukocytosis also are independent factors associated with outcome including cognitive outcome among survivors. In addition, several lines of evidence demonstrate a relationship between progression of infarction and worse neurologic outcome and elevated serum cytokines IL-1, IL-6, and TNFα. What is unclear is whether there is a causal relationship.
Cerebrospinal Fluid Inflammatory Markers
Cytokines and downstream activators of the inflammatory response can be measured in the CSF. This is better than brain biopsy but there are limitations to CSF sampling including: (1) sampling error when relative concentrations of proteins and molecules must diffuse into the CSF from a heterogeneously injured brain (i.e., the measured values likely reflect a weighted average from surrounding tissue and its proximity to the subarachnoid space); (2) CSF levels of individual cytokines may be related to their diffusion properties in fluids and distance from the source; (3) it is restricted to intermittent (e.g., daily) sampling; and (4) different half-lives of these inflammatory markers both in the CSF and collecting vials. In addition, it should be remembered that most cytokines act over short distances to effect local cells. It may therefore be desirable in some patients to obtain tissue and fluid closest to the injury, to allow for more specific sampling of brain inflammation.
Several clinical studies suggest that CSF and blood are functionally separate compartments when cytokine concentrations are measured. For example, Shiozaki et al. compared CSF and serum concentrations of several cytokines from patients suffering from multiorgan trauma and TBI to those with isolated TBI. The concentrations of the proinflammatory cytokines TNFα and IL-1 were greater in CSF than serum in those with isolated brain injury. In patients with multiorgan trauma, serum levels were greater than in CSF, whereas CSF concentrations of both proinflammatory and anti-inflammatory cytokines were similar to those with isolated CNS injury. There was an association between Injury Severity Score, an index of systemic injury burden, and tumor necrosis factor-α receptor 1 (TNFr1). Patients with elevated ICP had higher concentrations of CSF IL-1, IL-10, IL-Ira, and TNFr1 than those with normal ICP. These results suggest that CSF cytokine monitoring will more accurately reflect CNS pathology than serum cytokines.
Hydrocephalus that requires external ventricular drainage is common following SAH; hence CSF samples often are available. SAH has been found to cause an increase in cytokines IL-1, IL-6, transforming growth factor-β (TGFβ) and TNFα released from leukocytes in CSF and so monitoring the inflammatory response and in particular IL-6 maybe a useful tool to help guide care of SAH patients. However, in SAH patients who develop systemic inflammatory response syndrome (SIRS) ventricular CSF cytokine levels do not appear to correlate with serum levels of IL-1 and TNFα. In addition, although SIRS is associated with poor outcome, it may not always be associated with vasospasm. In general SIRS is more common in patients in poor clinical grade, and some but not all studies suggest it is associated with how an aneurysm is occluded.
IL-1 and IL-6 levels consistently have been observed to be greater in poor-grade than good-grade patients although an initial inflammatory response is observed in patients of all clinical grades. There also appears to be a relationship with DCI and inflammatory markers particularly in the CSF. For example, Mathiesen et al. analyzed CSF daily following SAH and found IL-1 to increase during delayed ischemic neurologic deficit (DIND). Elevated IL-1 and TNFα levels also correlated with poor outcome. Kwon and Jeon found that admission IL-6 concentration correlated with delayed ischemic deficits. SAH also appears to induce the production of nitric oxide metabolites, as inferred by the association with increased levels of nitric oxide breakdown products, nitrite and nitrate (NOx), in CSF. However, nitric oxide metabolites in CSF appear to be different from serum concentrations. In patients who develop vasospasm and DCI, CSF concentrations of NOx are greater between 2 and 8 days after aneurysm rupture.
In TBI, CSF cytokine concentrations are elevated. The highest concentrations usually are measured during the first 24 hours, after which there is a decline. However, there is a difference between CSF and serum concentrations of various cytokines. The difference between plasma and CSF levels of cytokines does not appear to be associated with BBB breakdown. This suggests that the cytokines are released by microglia, astrocytes, and neurons rather than simply reflecting “spill-over” from the blood. There are conflicting data on what cytokine levels in the CSF mean. Some studies show that IL-1 concentration in CSF is associated with unfavorable outcome following TBI. Other studies, however, suggest that peak CSF IL-6 levels are associated with improved outcome, IL-6 deficiency is associated with poor outcome, and up-regulation of IL-10 production may play a neuroprotective role by attenuating TNFα release.
Methods to Sample Cytokines and Monitor Neuroinflammation in Clinical Practice
Immune system cells and brain cells produce cytokines that act as intercellular signaling molecules. Depending on their concentration, cytokines can have toxic or trophic effects and can be pro- or anti-inflammatory. In addition several cytokines can have synergistic effects, and therefore a full understanding of the interactions between both deleterious and protective cytokines rather than just the absolute concentration of a particular cytokine may be needed to derive meaningful interpretation of clinical data. Several other factors make clinical interpretation of human cytokine data complex. First, each cytokine is produced during a defined time period and so results may depend on when the samples are obtained. Second, cytokines are produced in different amounts within different compartments—blood, brain, extracellular fluid (ECF), and CSF—and hence what is sampled will influence the findings. It appears that IL-1b and vascular endothelial growth factor (VEGF) levels are greater in brain ECF than in CSF, whereas IL-6, TNF, IL-8 and IL-1ra are greater in CSF than in microdialysate. Third, there are complex interactions of the immune system with the BBB and blood-CSF barriers that influence the findings. Finally, it is unclear whether it is the peak cytokine level, the area under the curve, or the specific cytokine production relative to other mediators that is most important to the biologic effect.
In clinical practice there are four basic approaches to examine the role of inflammatory mediators: (1) blood sampling (both arterial and jugular venous), (2) CSF sampling, (3) microdialysis, and (4) direct tissue sampling from ex vivo or postmortem tissue. In addition, PET studies may be used to examine microglial activation. Blood sampling is the easiest technique because it is readily available, can be easily repeated, requires no specialized equipment, and cytokines can be screened for with multiplex assay techniques. However, cytokines in arterial blood samples may indicate both intracranial and extracranial pathology. Paired arterial and jugular venous sampling can be used to help examine the relative contributions of the brain or the systemic inflammatory response. However, detection of a gradient is not always feasible because the absolute concentrations of some cytokines are close to the limit of sensitivity of the assay techniques.
External ventricular drainage when used provides a useful method to evaluate CSF inflammation because CSF production of cytokines is thought to represent brain production. However, the concentration of a cytokine may depend on how the CSF is drained (i.e., intermittently or continuously) and CSF production. In addition, it is unclear whether CSF drainage is a method to eliminate various cytokines (i.e., is “therapeutic”). This then raises the question of the biologic relevance of any immune mediator detected in the CSF.
Microdialysis continuously samples the brain interstitial space (see Chapter 36 ). This may be the most biologically relevant compartment because inflammatory mediators are secreted here and act on their surface membrane target receptors. It can be difficult to assay cytokines and inflammatory mediators using microdialysis because these proteins have a greater molecular weight than the metabolic intermediaries (glucose, lactate, pyruvate) commonly examined with this technique. Consequently cytokines diffuse across the microdialysis membrane at a slower rate, and a smaller proportion crosses into the catheter (i.e., the relative recovery is low). Several techniques can be used to address this, including use of catheters with larger molecular weight cutoff membranes (e.g., 100 kDa), addition of colloids (such as dextrans or albumin) to the perfusate, and use of sensitive multiplex techniques to assay the cytokines (e.g., the Luminex xMAP platform). In the laboratory setting cytokine binders (e.g., heparin) have been added to the perfusion fluid. This has not been used clinically because it is conceivable that heparin is lost from the perfusate into the ECF.
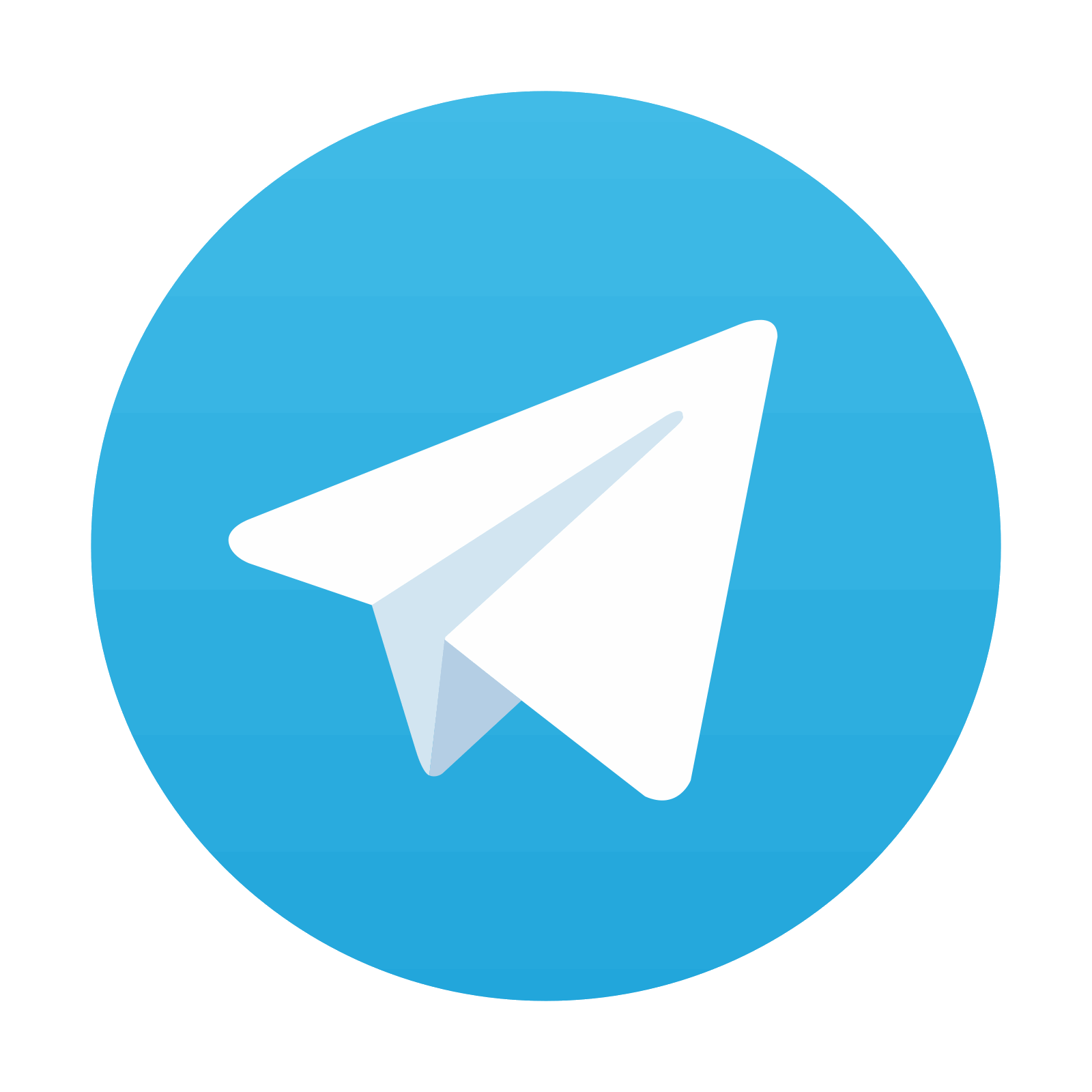
Stay updated, free articles. Join our Telegram channel
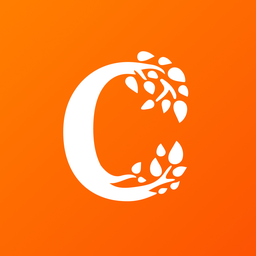
Full access? Get Clinical Tree
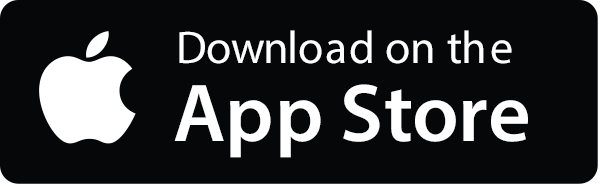
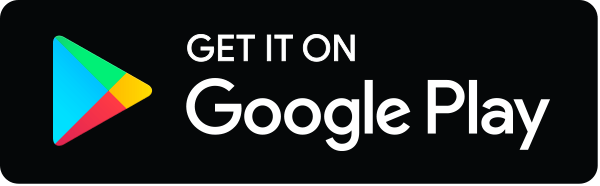