Chapter 142 Monitoring Techniques for Evaluating Suspected Sleep-Disordered Breathing
Abstract
Definitions
Abnormal breathing during sleep can take several different forms, arises from differing etiologies, and goes by many names, including sleep apnea, sleep apnea–hypopnea syndrome, sleep-disordered breathing (SBD), sleep-related breathing disorder (SRBD), periodic breathing, Cheyne-Stokes respiration, and hypoventilation (Box 142-1). SRBDs associated with neurologic lesions are described elsewhere in this volume. Some terms distinguish between different types of sleep-related breathing impairments; others are synonymous. SDB events include episodes of apnea, hypopneas, respiratory effort–related arousals, oxygen desaturations, and snore arousals.
Box 142-1 Sleep-Related Breathing Events, Disorder Classifications, and Parameters
Sleep-disordered breathing severity can be based on a clinical dimension (e.g., sleepiness), event frequency (e.g., number of events per hour), or magnitude of the consequence (e.g., degree of oxyhemoglobin desaturation). Table 142-1 provides examples for dimensionally classifying severity of SDB. There is no general agreement about assigning severity descriptors to indices of SDB; however, two schemes are commonly used. In the first (the liberal) classification, an apnea–hypopnea index (AHI) between 5 and 15 is mild, between 15 and 30 is moderate, and greater than 30 is severe. In the second (the conservative) classification, an AHI between 10 and 20 is mild, between 20 and 50 is moderate, and greater than 50 is severe.1
Methods to Detect Airflow
In general, airflow cessation or near cessation for 10 seconds or more constitutes a sleep apnea episode. Although the American Academy of Sleep Medicine (AASM) manual recommends a thermal sensor to document apnea and a nasal pressure sensor to detect hyponea,2 other methods provide reliable assessment. Occasionally, unidirectional occlusion occurs, with no airflow during attempted inspiration but tiny puffs of expiration. Fully quantitative airflow determination requires pneumotachography. Alternatively, a body box could be used; however, such an approach is unsuitable for sleep studies. Semiquantitative measures are attainable using calibrated inductance plethysmography; however, most clinical evaluations rely on qualitative nasal-oral thermography and nasal pressure to minimize the patient’s discomfort, reduce costs, and simplify acquisition of data. Airflow can also be measured qualitatively by detecting chemical differences between ambient and expired air (e.g., capnography).
Pneumotachography
Pneumotachography accurately and quantitatively measures airflow volume. The patient usually wears a facemask, the equipment can be bulky and large, and the procedure is usually uncomfortable. Therefore, its routine use for overnight assessment of SDB is problematic. In awake subjects, such invasive devices can increase tidal volume and reduce respiratory rate. Nonetheless, some positive airway pressure machines have built-in pneumotachographs and can be used to monitor airflow and ventilation. Cardiogenic oscillations can also be detected to provide a possible marker for central apnea.3
Nasal Airway Pressure
During inspiration, airway pressure is negative relative to atmosphere. By contrast, expiration produces a relatively positive pressure in the airway. The resulting alteration in nasal airway pressure can provide a surrogate estimate of airflow and correlates favorably with pneumotachographically recorded signals.4 The nasal pressure signal also offers greater sensitivity for detecting subtle flow limitations than nasal–oral thermography (Fig. 142-1) when persons breathe through the nose.5 Airflow limitation manifests as pressure trace plateauing during inspiration. A direct current (DC) amplifier provide optimal interface; however, long time-constant alternating current (i.e., a very slowly coupled signal) can suffice. By contrast, rapid coupling can create artifact (Fig. 142-2).
Expired Carbon Dioxide Sensing
During central apnea, a low-volume catheter system set at its most rapid response time can show cardiogenic oscillations in the CO2 signal. These oscillations result from small volume displacements caused by the beating heart.6 These heartbeat-synchronized oscillations signify a wide-open upper airway (Fig. 142-3).
Infants and children with upper airway obstruction can severely hypoventilate during sleep without observable apnea or hypopnea. Measuring expired CO2 provides evidence for hypoventilation not detectable using thermistors or thermocouples.6
Cautionary Note Concerning Qualitative versus Quantitative Airflow Measures
The vast majority of clinical sleep evaluations use qualitative measures of airflow for detecting SDB events. Extreme care must be taken when classifying respiratory activity because a patient’s airway can completely occlude during inspiration but release small puffs on expiration (detectable by thermistor or CO2 analyzer). Such events are erroneously categorized as hypopneas or even normal (unobstructed breathing). Figure 142-4 illustrates the problem. Airflow is recorded simultaneously with a CO2 analyzer and a pneumotachograph. During the obstructive apnea, periods of expiratory airflow occur (recorded by the pneumotachograph and the CO2 analyzer) in the absence of inspiratory flow (obvious in the pneumotachograph recording and unclear in the CO2 recording). Without the information from the pneumotachograph, the recording from the CO2 analyzer would be interpreted as evidence of uninterrupted inspiratory and expiratory airflow.
Methods to Detect Respiratory Effort
Rib Cage and Abdominal Motion
During normal breathing, the major inspiratory muscles produce rib cage expansion and a downward movement of the diaphragm. These movements cause the pressure around and in the lung to become negative (relative to atmospheric pressure). The pressure gradient between ambient air and the lung draws air through the airways into the alveoli. Thus, a change in lung volume is the sum of the volume changes of the structures surrounding the lungs, the rib cage, and the abdomen.7 Other respiratory muscles (e.g., intercostal, sternocleidomastoid) also play a role in stabilizing the thoracic cage. Some clinicians erroneously interpret the abdominal and rib cage motion changes as implying separate activities of abdominal and thoracic respiratory muscles, but this is not the case. Virtually all the changes in abdominal and rib cage volumes (including paradoxical motion) can be explained by changes in the respiratory muscles directly inserting onto the thoracic cage. Paradoxical motion of the rib cage and abdomen can result from several changes, including loss of tone of the diaphragm, loss of tone of the other respiratory muscles, or upper airway obstruction (complete or partial). The mechanisms underlying this asynchronous motion of rib cage and abdomen are described in Box 142-2. Regardless of the pattern or its underlying mechanism, rib cage and abdominal movement reflect effort to breathe.
Respiratory Muscle Electromyography
One of the older techniques for detecting respiratory effort entail recording intercostal muscle electromyographic (EMG) activity (Fig. 142-5). These uncalibrated recordings are made using standard surface electrodes placed in pairs in the intercostal space on the right anterior chest. Attaining an optimal signal requires practice, patience, and skill; recordings are prone to artifact, especially artifact from the electrocardiogram (ECG). Intercostal EMG recordings, when recorded properly, can be extremely valuable for differentiating central, obstructive, and mixed SBD events. Furthermore, although signals are not calibrated, cascading increases in respiratory effort are readily apparent from recordings.
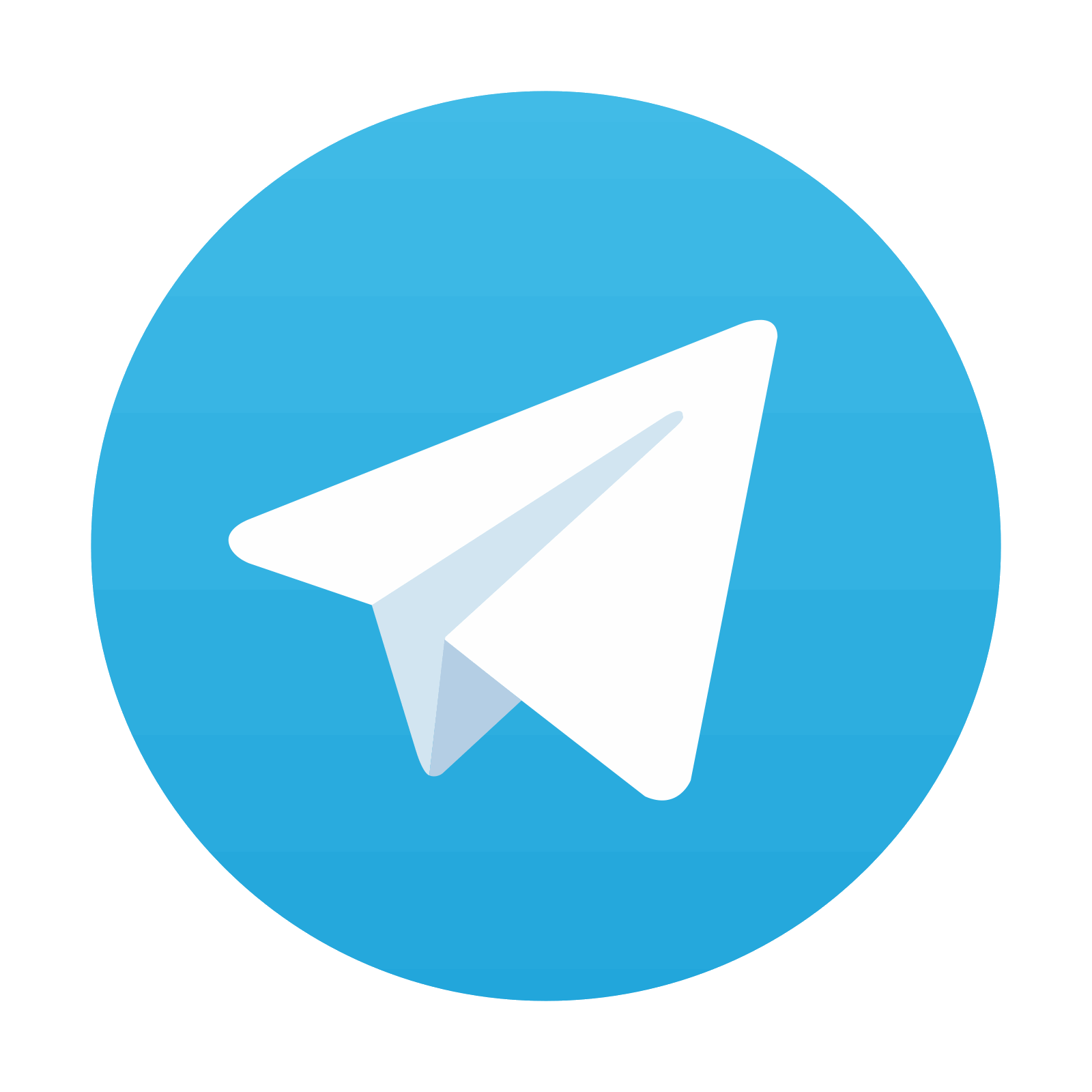
Stay updated, free articles. Join our Telegram channel
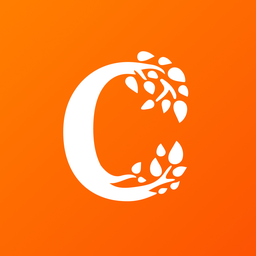
Full access? Get Clinical Tree
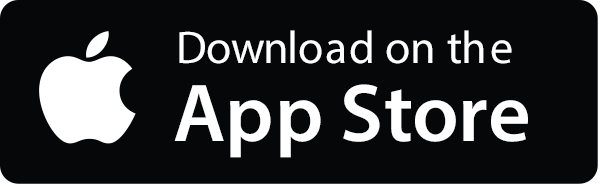
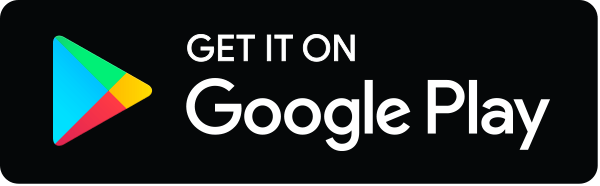