Introduction
Need for Anesthesia and Monitoring During Surgery
Monitoring is an essential component of patient care in the operating room, and standard monitoring mandated by the American Society of Anesthesiology includes blood pressure, electrocardiogram, pulse oximetry, end-tidal CO 2 (Et CO 2 ), and temperature. However, for the neurosurgical patient, either the surgical procedure or the patient’s pathology may necessitate specific monitoring of the central nervous system. The interpretation of these monitored variables is potentially subjected to the following influences: (1) pathophysiologic changes (related to the patient’s disease or surgical manipulation), (2) physiologic influences (related to changes that are within “physiologic range”), and (3) anesthetic influences (changes that are anesthesia related). Pathophysiologic interpretation of these monitors is covered elsewhere in this textbook, and “physiologic changes” are relatively minor. The focus of this chapter is on the potential interactions and influence of anesthetic agents on these monitors.
Common Anesthetic Techniques
Although most neurosurgical procedures are performed under general anesthesia, some procedures such as functional neurosurgery require either a cooperative patient or one with intrinsic cortical or subcortical electroencephalographic activity unaltered by any central nervous system depressants. In general the anesthetic techniques can be classified into three categories:
- 1.
General anesthesia. The patient is rendered unconscious in the conventional manner with the use of hypnotic/potential inhaled or intravenous agents/narcotics with or without neuromuscular blocking agents.
- 2.
Regional anesthesia. A field block or regional block with local anesthetic with and without use of sedative is used. This has limited application in neurosurgical procedures, and is primarily used for carotid endarterectomy or functional neurosurgery where sedatives usually are omitted.
- 3.
Monitored anesthesia care. Only light sedation is used in these cases to supplement local anesthetic at the surgical site. This technique may apply to epilepsy surgery or tumor surgery that encroaches on eloquent areas where patient cooperation is required. It also is used commonly in neuroradiologic procedures, some endovascular procedures, or peripheral nerve surgery.
Anesthetic Agents
Anesthetic agents include hypnotics, analgesics, neuromuscular blockers, and other adjuncts. Their effects on interpretation of monitors are mediated by (1) direct effect—the specific intrinsic influence of each anesthetic agent on the physiologic activity of the brain (i.e., blood flow, metabolism, or function), or (2) indirect effect—changes in monitored variables secondary to decreased perfusion of the brain secondary to the systemic/cerebral effects of the anesthetic. The influence is unique to the anesthetic agent and to the monitored variable, and is discussed under the monitored modalities later. The common anesthetic agents in current practice include the following:
Hypnotics
Inhaled agents: desflurane, sevoflurane, isoflurane, halothane, and nitrous oxide (N 2 O)
Intravenous agents: propofol, thiopental, benzodiazepines, ketamine, and etomidate
Analgesics
Morphine and other synthetic opiates, nonsteroidal anti-inflammatory drugs, gabapentin, and pregabalin.
Neuromuscular blockers
Short-acting agents, such as succinylcholine; intermediate-duration agents, such as rocuronium and vecuronium; and long-acting agents, such as pancuronium
Other adjuncts
Neuroleptics and α-2 agonists, such as dexmedetomidine
Monitors and the Influence of Anesthetic Agents
Cerebral Blood Flow and Surrogates
Continuous direct measurement of cerebral blood flow (CBF) continues to be an elusive goal, although laser Doppler and thermodilution flow meters are available. They can be difficult to use and are not widely used during surgical procedures. Anesthetic agents do not directly influence the function of these monitors, but can alter CBF (see Transcranial Doppler Ultrasonography ).
Jugular Venous Oximetry
Jugular venous oxygen saturation reflects the balance between CBF and cerebral oxygen metabolism. Thus a higher jugular venous oxygen saturation (>70%) suggests flow in excess of metabolic need, and conversely, a low saturation (<50%) indicates increased extraction and is suggestive of ischemia. Anesthetic agents can influence this balance between flow and metabolism. Inhaled agents tend to result in higher jugular venous saturation, whereas intravenous agents such as propofol result in lower saturation. More important than the anesthetic influence is the cerebrovascular response to change in carbon dioxide (CO 2 ) tension; thus hyperventilation can result in low jugular venous saturation, and hypoventilation may have the opposite effect. It is generally considered prudent to maintain jugular venous saturation greater than 50%. Although not a widely adopted practice, the use of jugular venous oximetry is considered a useful intraoperative monitor for craniotomy at some centers where hyperventilation is used.
Intracranial Pressure and Cerebral Perfusion Pressure Monitor
Monitoring of intracranial pressure (ICP) provides useful information about the status of the intracranial compartment, and just as importantly the net perfusion pressure to the brain (cerebral perfusion pressure = mean arterial pressure − ICP). Anesthetic agents do not per se interfere with the measurement of ICP, but they can alter ICP by causing vasodilation or vasoconstriction. In general, inhaled agents cause either no change or a dose-related increase in CBF, whereas intravenous agents such as propofol cause vasoconstriction and so a reduction in ICP (ketamine is an exception). The Prx index, that is, the moving correlation coefficient between mean ICP and mean arterial blood pressure, can provide information about autoregulation. However, the effects of anesthetics on Prx remain unclear.
Transcranial Doppler Ultrasonography
Although not widely used in the operating room because of technical and labor-intensive demands, transcranial Doppler (TCD), when properly applied, can be a useful continuous monitor of relative changes in CBF.
TCD is a noninvasive technique that assesses blood flow velocity (BFV) and direction in the cerebral arteries by means of a pulsed ultrasonic beam. Aaslid et al first described the TCD technique in 1982. Today there are multiple clinical applications for TCD that are described in detail in Chapter 30 . This chapter addresses the use of TCD as an intraoperative monitor and effects of anesthetic agents on TCD.
TCD has the ability to noninvasively assess local CBF velocities in the major intracranial arteries. This determination of cerebral blood velocities allows for an indirect assessment of CBF, and TCD can quantitatively measure relative changes in CBF. This property makes TCD an appealing intraoperative monitor, in that maintenance of adequate cerebral perfusion is a goal in any operative procedure.
Bilateral insonation of the middle cerebral arteries (MCAs) through the temporal bone (temporal window) is the most commonly used intraoperative application of TCD. This allows for comparison of blood flow velocities between hemispheres, emboli monitoring, assessment of cerebral autoregulation, and CO 2 reactivity. However, TCD has several limitations that prevent routine application of it as an intraoperative monitor:
- 1.
In neurosurgical patients there is limited access to acoustic windows required for accurate flow velocity measurements because of the surgical incision sites and the sterile surgical field during a craniotomy. This limitation, however, may not apply during a carotid endarterectomy.
- 2.
Patient positioning complicates placement of the ultrasound transducers in stable positions. For reliable, continuous assessment of cerebral BFV, TCD probes require a constant insonation angle (CBF velocity is proportional to the cosine of the angle of insonation). This requires the use of a fixation device, such as the LAM-Rack, to stabilize the ultrasound transducers, because handholding techniques or intermittent measurements are unreliable.
- 3.
About 20% of patients have inadequate acoustic windows because of temporal bone hyperostosis.
Despite its limitations, TCD can be a valuable intraoperative monitor. It is particularly useful during carotid endarterectomy, in which it can be more accurate than near infrared spectroscopy (NIRS), stump pressure and somatosensory evoked potentials (SSEP) in patients at risk for intraoperative cerebral hypoperfusion or emboli. TCD also allows for assessment of postoperative embolic phenomenon and hyperperfusion. Both of these conditions are associated with postoperative neurologic insult and stroke.
Anesthetic Effects on Blood Flow Velocity
Unlike other neurophysiologic monitoring techniques, TCD is relatively resistant to interference from anesthetic agents. However, changes observed in CBF under anesthesia may reflect physiologic changes of the patient associated with anesthetic influence. Consequently, TCD has been used to study neurophysiologic effects of anesthetic agents. Table 9.1 summarizes these anesthetic physiologic effects measured by TCD .
Anesthetic Agent | CBFV | Autoregulation | CO 2 Reactivity | References |
---|---|---|---|---|
N 2 O | ↑ | Impaired | Preserved | |
Isoflurane (0.5-1.5 MAC) | ↗ | Preserved at 1 MAC, ↘>1MAC | Preserved at 1 MAC | |
Desflurane (0.5-1.5 MAC) | ↗ | Preserved at 1 MAC, Impaired at 1.5 MAC | Preserved at 1 MAC | |
Sevoflurane (0.5-2 MAC) | ↗ | Preserved at 1.5 MAC | Preserved at 2 MAC | |
Midazolam (0.15 mg/kg bolus) | ↔ | |||
Thiopental (5 mg/kg bolus) | ↓ | |||
Propofol (2 mg/kg bolus) | ↓ | |||
Propofol (25-200 mcg/kg/min) | ↘ | Preserved | Preserved | |
Etomidate (0.3 mg/kg bolus) | ↓ | Preserved | ||
Ketamine (1.5 mg/kg bolus) | ↑/↔ | Preserved | ||
Dexmedetomidine (various doses) | ↘ | Impaired | Impaired | |
Fentanyl (various doses) | ↔ | Preserved | ||
Remifentanil (various doses) | ↔ | Preserved | Preserved | |
Sufentanil (0.1-0.2 vs 6 mcg/kg) | ↔/↘ |
Inhalational Anesthetics
CBF-metabolism coupling is generally preserved during inhalation anesthesia, and has been extensively studied using TCD, because changes in cerebral blood volume reflect corresponding CBF changes. Although flow-metabolism coupling is preserved with volatile anesthetics ; the net effect of a volatile anesthetic on CBF depends on the balance between the agent’s direct vasodilatory effect and flow-metabolism coupling-mediated vasoconstriction.
Sevoflurane is a commonly used volatile anesthetic during neurosurgical procedures because it has relatively low blood-gas solubility that allows rapid titration and emergence, and has favorable cerebral hemodynamic effects compared with other volatile anesthetics. Sevoflurane, similar to other volatile anesthetics, is a central nervous system depressant that can decrease the cerebral metabolism. This depressant effect causes TCD-measured cerebral BFV to decrease secondary to flow-metabolism coupling. As concentrations of sevoflurane are increased, TCD flow velocities tend to increase, but to a lesser extent than other volatile agents ; this reflects the influence of the intrinsic cerebrovasodilatory effect of sevoflurane and can result in luxury perfusion states with concentrations greater than 1.5 minimum alveolar concentration (MAC). It is unusual that clinical concentrations are required above this level. Furthermore, at 1.5 to 2 MAC, cerebral autoregulation mechanisms and cerebral reactivity to CO 2 are well preserved.
Desflurane has the lowest blood-gas solubility coefficient of the halogenated ether anesthetics, making it desirable for use in neuroanesthesia because it facilitates a rapid emergence. However, cerebral physiologic effects of desflurane are not as favorable as for sevoflurane. Desflurane causes a dose-dependent increase in cerebral BFVs; this takes effect at much lower MAC values than for sevoflurane. To reduce the luxury perfusion, increased cerebral blood volume and potential increase in ICP that can be caused by desflurane, concentrations should be limited to 1 MAC. Cerebral autoregulation and CO 2 reactivity are preserved at this clinically relevant concentration.
Isoflurane has a long history of use as a neuroanesthetic agent. Similar to other volatile agents, isoflurane has intrinsic dose-dependent cerebral vasodilatory properties. When unopposed by flow-metabolism coupling-mediated vasoconstriction, isoflurane, in concentrations of 0.5 to 1.5 MAC, causes significant increases to cerebral BFVs. The effect of isoflurane on cerebral autoregulation is dose dependent, and this homeostatic mechanism is preserved up to concentrations of 1 MAC, beyond which it is impaired. However, this depression of cerebral autoregulation can be restored with hyperventilation. CO 2 reactivity is maintained at 1 MAC.
N 2 O is most commonly used as an adjuvant to other anesthetic agents, because hyperbaric conditions are required to reach anesthetic concentrations with N 2 O alone. N 2 O is a potent cerebral vasodilator, and results in increased cerebral BFVs. N 2 O also increases cerebral metabolism and can increase CBF through vasodilation. Furthermore, cerebral autoregulation is impaired with N 2 O. Together these factors make N 2 O an undesirable agent in patients with limited intracranial compliance. However, cerebrovasomotor reactivity to CO 2 is preserved.
Intravenous Anesthetics
Propofol is commonly used as an anesthetic induction agent and to maintain anesthesia. It is easily and rapidly titratable and well tolerated for use during evoked potential monitoring, and its cerebrovascular effects are favorable in the neurosurgical patient. Propofol causes a dose-dependent decrease in cerebral BFVs, supporting its preservation of flow-metabolism coupling. Propofol also has direct cerebral vasoconstricting properties that contribute to the observed BFV decreases. Cerebral autoregulation and CO 2 reactivity are well preserved.
Thiopental is a barbiturate anesthetic, which like propofol, can be used for both induction and maintenance of anesthesia. However, thiopental’s pharmacodynamic profile can be undesirable in the neurosurgical patient in that it delays emergence. Nevertheless, thiopental is frequently used as a neuroprotective agent during aneurysm surgery (e.g., during temporary arterial occlusion), because it decreases cerebral metabolism with the induction of burst suppression. Induction doses of 5 mg/kg decrease cerebral BFV.
Ketamine is an intravenous anesthetic agent with dissociative anesthetic properties. It has a controversial history in the neurosurgical patient in that older evidence showed ketamine could increase CBF velocities, cerebral metabolism, and ICP. Ketamine increases cerebral BFV during induction, but as an adjuvant to an anesthetic under controlled ventilation, ketamine does not increase cerebral BFV. When used with propofol as part of a total intravenous technique, ketamine preserves cerebral autoregulation.
Dexmedetomidine is a selective α-2 agonist used as a sedative in intensive care units and in the operating room for procedures such as awake craniotomies and carotid endarterectomies. It has sedative properties with minimal respiratory depression that makes it appealing for these applications. Dexmedetomidine decreases cerebral BFVs in a dose-dependent manner, which appears to result from decreased cerebral metabolic rate with preserved flow-metabolism coupling. However, at sedative doses, dexmedetomidine may cause a slight decrease in both dynamic cerebral autoregulation and CO 2 reactivity.
Etomidate is an imidazole derivative intravenous anesthetic agent commonly used as an induction agent, particularly in trauma patients or those with limited cardiac reserve, because of its hemodynamic stability. Induction doses of etomidate lead to decreased middle cerebral artery BFVs. CO 2 reactivity is preserved during etomidate infusion ; however, infusions are not recommended because there may be adrenal suppression and propylene glycol toxicity.
Midazolam is a commonly used intravenous benzodiazepine. It is used frequently as a preoperative anxiolytic or as an infusion in intensive care units as a sedative. There is conflicting evidence on the effects of midazolam on cerebral BFVs. In one study midazolam at 0.15 mg/kg with fentanyl did not affect MCA BFVs. In other studies, however, at a much lower dose of 20 and 40 µg/kg, cerebral BFVs decreased about 20% but returned to baseline in 5 minutes.
Opioids are used frequently during neurosurgery for multiple reasons: to blunt sympathetic responses to stimulation, to treat pain, and as adjuvants to help reduce the doses of other anesthetic drugs. In clinically relevant concentrations with controlled ventilation, opioids have limited effects on cerebral physiology. Fentanyl and remifentanil do not significantly change cerebral BFVs. CO 2 reactivity and cerebral autoregulation also remain intact. Low doses of sufentanil (0.1 to 0.2 µg/kg) do not affect cerebral BFVs; however, increasing doses can decrease cerebral BFV in a dose-dependent fashion.
Cerebral Function
Electroencephalography (Unprocessed and Computer Processed)
Electroencephalogram (EEG) activity can be monitored intraoperatively for a variety of reasons, such as to detect cerebral ischemia or to localize seizure activity. However, all EEG correlates or derivatives are influenced by anesthetic agents. Both intravenous anesthetics and inhalation anesthetics cause dose-related depression that ultimately may result in electrical silence. Low-dose inhalation agents can cause initial stimulation with an increase in EEG activity in the alpha band. With progressive increase in depth, there is an increase in delta wave, and disappearance of beta and alpha bands. With further increase in anesthetic depth, burst-suppression progressing to electrical silence will occur. The dose-response curve is different among the inhaled anesthetics. Isoflurane will cause burst-suppression at 1.5 MAC and electrical silence at 2 MAC, whereas sevoflurane causes burst-suppression at 2 MAC and electrical silence at 2.5 MAC. There also is an age-anesthetic effect interaction, with lower anesthetic doses needed to achieve burst suppression in older patients. N 2 O paradoxically can cause an increase in EEG activity when added to an inhalation anesthetic. Seizure activity also has been reported with most anesthetic agents. Even though some agents have a higher propensity to cause epileptogenic activity, the clinical significance in unclear. For example, inhalation anesthetics including sevoflurane and enflurane can cause epileptogenic spikes in healthy patients without a history of epilepsy. Seizure activity can interfere with monitoring of computer-processed EEG such as the Bispectral Index. Intravenous agents in general do not cause stimulation and slow waves develop early. Both thiopental and propofol at increasing doses will result in burst suppression pattern followed by electrical silence. This is used often for brain protection during periods of potential ischemia.
Because anesthetic agents can have a profound influence on EEG, anesthetic depth must be maintained at a relatively steady state to reliably monitor cerebral ischemia, to allow meaningful interpretation of changes. These agents and benzodiazepines must be avoided during functional surgery when mapping of electrical activity or epileptic foci is important. For both deep brain stimulator implantation and resection of epileptic foci in patients who are otherwise awake, dexmedetomidine as the sole sedative agent has been used with some success, without interfering with electrophysiologic recording.
Evoked Potentials
Multimodality evoked potentials increasingly are used intraoperatively to monitor and help preserve integrity of the central nervous system structures placed at risk by the surgical procedure. Anesthetic agents have unique and profound influence on evoked potentials, and these effects must be understood to allow meaningful recording and interpretation of these signals. The modalities include SSEP, motor evoked potentials (MEP) and cranial nerves with motor components (V, VII, IX, X, XII).
Somatosensory Evoked Potential Monitoring
An important clinical decision when using SSEP monitoring is the choice between inhalational and intravenous anesthetic. Even though SSEPs are not degraded to the same extent as MEPs by inhalational anesthetics, they exhibit a dose-related increase in latency and decrease in amplitude under volatile anesthesia. This is particularly true for cortical SSEPs that involve multiple synapses. Brainstem and spinal components are relatively resistant to anesthetic effects. When volatile agents are used, the dose should be kept below 1 MAC to maintain SSEP signal quality. This may be facilitated by the coadministration of potent opiates, which do not affect signal quality. The addition of N 2 O typically is avoided, because this further degrades the signal. In addition, the anesthetic concentration should be kept constant during critical periods to reduce anesthetic influence on the interpretation of changes. The use of intravenous anesthetics, such as propofol, thiopental, or midazolam infusion, often results in better signal quality than with use of volatile agents. Ketamine and etomidate, in particular, have been reported to increase the amplitude of SSEPs, although the effect that this has on sensitivity and utility remains a matter of speculation. Regardless of the anesthetic regimen, it is important to avoid bolus doses or rapid changes in anesthetic depth during key portions of an intervention, such as vessel occlusion or manipulation of the spine, to avoid confounding influences on signal integrity at a critical time when the monitoring modality is most useful.
Other factors, such as blood pressure, temperature, and patient position, may influence evoked potential monitoring. Nerve injury associated with patient position, such as crush injury at pressure points, or traction injury must be considered. A decrease in blood pressure may also alter SSEPs and even mild hypothermia can increase latency and decrease the amplitude of evoked potentials because thermoregulatory behaviors and physiologic responses are blunted in the anesthetized patient.
Brainstem Auditory Evoked Potential Monitoring
Brainstem auditory evoked potentials (BAEPs) are resistant to anesthetic effects and can be recorded with virtually any anesthetic technique. Inhalation agents can increase the latency but do not abolish BAEPs. The effects of anesthetic agents on BAEP and SSEP are summarized in Table 9.2 .
BAEP | SSEP | |||
---|---|---|---|---|
Latency | Amplitude | Latency | Amplitude | |
Thiopental Low dose High dose | ||||
↔ | ↔ | ↑ | ↓ | |
↑ | ↓ | ↑ | ▾ | |
Propofol | ↑ | ↔ | ↑ | ↔ or ↓ |
Dexmedetomidine | ? | ? | ↔ | ↔ |
Midazolam | ? | ? | ↔ | ↓ |
Etomidate | ↔ | ↔ | ↑ | ▴ |
Ketamine | ↔ | ↔ | ↔ | ▴ |
Fentanyl | ↔ | ↔ | ↑ | ↓ |
Remifentanil | ↔ | ↔ | ↔ | ↔ |
Halogenated volatile agents 0.5 Mac >1 Mac | ||||
↑ | ↔ | ↔ or ↑ | ↔ or ↓ | |
▴ | ▾ | |||
Nitrous oxide | ↔ | ↔ | ↔ | ▾ |
Motor Evoked Potentials
MEPs are electrical potentials monitored along the motor pathway after stimulation of the motor cortex or spinal cord. MEPs are elicited by direct or transcranial stimulation with either electrical (TcEMEP) or magnetic (TcMMEP) techniques. Responses can be recorded over the spinal cord from electrodes placed in the epidural space (spinal D waves or epidural MEP), along peripheral motor nerves (neurogenic MEP), or as compound muscle action potentials (CMAPs) over muscle (myogenic MEP). Myogenic MEPs are large amplitude biphasic potentials in response to motor unit depolarization, and so, unlike SSEPs, signal averaging is not required. This allows for near instant motor pathway feedback. TcMMEPs are exquisitely sensitive to anesthetic agents and so are unsuitable for intraoperative monitoring.
The entire motor pathway from cerebral motor cortex through brainstem, corticospinal tracks, anterior horn, peripheral nerve, and neuromuscular junction to skeletal muscle can be assessed using transcranial stimulation with myogenic MEP monitoring. Motor pathway injury can result from ischemia, or disruption, retraction, compression, or distraction of neural tissue. Each of these insults can cause functional alterations that may be neurophysiologically evident. MEPs therefore are an appealing intraoperative monitor and are applicable to any surgical procedure which places the motor pathway at risk for injury: craniotomy, spinal surgery, or thoracoabdominal aortic aneurysm repair. Intraoperatively, MEPs are usually monitored with SSEPs.
MEPs are useful to monitor for ischemia, particularly of the anterior horn cells (alpha motor neurons), which are thought to be most sensitive to ischemic insults. Many publications describe the use of MEP for intraoperative monitoring, although there are no clearly defined thresholds for latency or amplitude changes that correlate with risk of motor pathway injury. In general, latency is robust to anesthetic or surgical insults; therefore amplitude changes are assessed more commonly. The threshold considered concerning for injury is the complete loss of signal rather than a fixed percentage decrease in amplitude (e.g., 50%). Other authors consider the increase in stimulation energy required to reproduce baseline signals as the marker for injury. The safety of MEPs is well known. However, induction of seizures is a potential risk with TcEMEPs.
Anesthetic Effects
MEPs are sensitive to anesthetics; therefore a tailored approach is required to select the most appropriate anesthetic regimen. Transcranial magnetic stimulation–generated MEPs are more sensitive to anesthetics than transcranial electrical (TcE) stimulation, and usually are abolished with induction, regardless of the agents used. TcE stimulation therefore is the technique used exclusively during intraoperative monitoring. Transcranial magnetic stimulation currently has a very limited role in the operating room. Early work with TcEMEPs showed that single-pulse signals were extremely sensitive to volatile agents even at subclinical doses. Subsequently, multipulse stimulation techniques have been shown to improve both low-amplitude baseline signals and MEP signals depressed by anesthesia ( Fig. 9.1 ). Table 9.3 summarizes how different anesthetic agents affect multipulse TcEMEPs, which is the current standard during intraoperative monitoring. “Anesthetic fade,” a time-dependent decrease in MEP signal amplitude proportionate to the length of surgery regardless of the anesthetic regimen can occur. Increasing the stimulation energy may attenuate anesthetic fade, but increases the risk for potential false-positive signal changes.
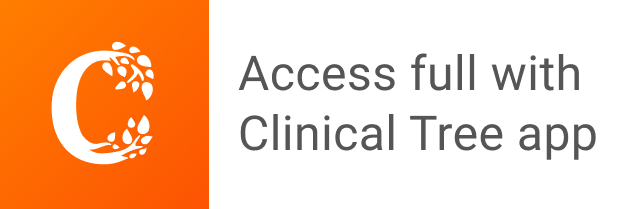