Emotions activate physiologic, cognitive, and behavioral outputs that facilitate adaptive responses to salient external and internal stimuli.
A crucial emotion, important in several mental disorders, is fear. The neural circuitry of fear is well understood because fear can be reliably elicited in animal models, and because its effects can readily be measured.
Information about threatening stimuli is transmitted from the thalamus and cerebral cortex to the amygdala where it is processed; neurons carrying fear-related information then project to diverse downstream sites in the brain responsible for adaptive responses.
Anxiety, a state characterized by arousal, vigilance, physiologic preparedness, and, in humans, negative subjective states, may share some critical circuits with fear.
Because of the evolutionary conservation of key neural circuits, animal research on fear has relevance to humans. Several anxiety disorders may involve abnormal regulation of amygdala-based fear circuitry.
Mood disorders are divided into unipolar disorders, which are characterized by depression only, and bipolar disorder, which is diagnosed if the person has ever had an episode of mania.
Mood disorders are influenced by both genes and environment, with genes playing a greater role in bipolar than in unipolar disorders.
Animal models to study mood regulation and mood disorders are far from perfect. However, in concert with human experiments involving functional neuroimaging, studies of postmortem brain tissue, and clinical trials of deep brain stimulation, a picture of mood-regulating circuits is emerging.
A subset of individuals with major depression exhibit excessive activation of the hypothalamic–pituitary–adrenal axis; thus, certain cases of depression share some physiologic mechanisms with chronic stress.
Most of the effective pharmacologic treatments for depression act on protein targets within monoamine synapses, generally enhancing neurotransmission by norepinephrine, serotonin, or both. A similar role for dopamine is postulated but unproven.
Although the initial molecular targets of antidepressants are well characterized, the actual mechanism of action is not understood. The several-week latency of onset of therapeutic effects suggests that slowly developing adaptive responses to initial enhancement of monoamine neurotransmission are required for efficacy.
Current research aimed at developing more effective antidepressants with novel mechanisms of action is focused on several forebrain regions and the actions of numerous neurotransmitter systems, neurotrophic factors, cytokines, intracellular signaling pathways, and transcriptional regulatory mechanisms.
The discovery of ketamine, an NMDA glutamate receptor antagonist, as a rapidly acting antidepressant has opened up new avenues in depression and antidepressant research.
Emotions are critical to survival and represent transient physiologic, cognitive, and behavioral outputs that constitute adaptive responses to survival-relevant or otherwise salient stimuli. Mood is a term used to characterize the predominant emotional state over time. Moods (eg, happy, sad, irritable) interact bidirectionally with emotional responses to particular stimuli.
Although there are many subtle forms of emotion, they may be divided into two broad categories by their valence. Negative emotions, such as fear, are elicited under normal circumstances by stimuli that connote danger, pain, or other noxious conditions and generally lead to avoidance, escape, or protective responses. Positive emotions are elicited by stimuli that connote food, safety, comfort, or reproductive opportunities and lead to approach behaviors.
Many common human diseases produce pathologic emotional states that may be transient or enduring. Although most individuals with these disorders can be treated, at least in part, with available pharmacologic agents, their mechanisms of action are generally not deeply understood. For example, the initial molecular targets of anxiolytic (antianxiety) and antidepressant drugs have been determined, but the mechanisms by which these drugs ultimately produce their therapeutic effects have yet to be adequately characterized.
Emotion processing circuits in the brain appraise the valence and potency of stimuli and activate appropriate responses. The neural substrates of emotion involve, in part, evolutionarily old brain regions lying deep to the neocortex 15–1, including the amygdala, hippocampus, parahippocampal gyrus, and cingulate gyrus. These structures have strong interconnections with the hypothalamus, ventral striatum (nucleus accumbens), and the orbital and medial prefrontal cortex. Although the older term “limbic system” (comprising an inexact collection of forebrain structures) is still in use, suggesting some central processor for emotion, it is clear based on lesion studies in animals, strokes, and other brain injuries in humans, and functional neuroimaging that different emotions (eg, fear, disgust, trust) utilize distinct circuits involving limbic structures, frontal regions of cortex, and the hypothalamus.
15–1
Limbic regions involved in emotion and mood. A. A ring of tissue (limbus) composed of the hippocampus and amygdala as well as the cingulate and parahippocampal gyri encircles the upper brainstem. It consists of evolutionarily older forms of cortex than the six-layered neocortex. This ring has been called the limbic system, but is no longer thought to represent a single-purpose system involved in emotion. Rather “limbic” structures play important roles in diverse circuits that interact with the prefrontal cortex and the hypothalamus. B. Multiple views of the prefrontal cortex and related areas. Panel 1: View from the bottom of the brain showing the ventromedial (pink) and orbital (green) prefrontal cortex. Panel 2: Dorsolateral prefrontal cortex (purple). Panel 3: Hippocampus (bottom, pink) and amygdala (top, stippled pink). Panel 4: Anterior cingulate gyrus (yellow), a region of medial prefrontal cortex. Brodmann area 25, a target for deep brain stimulation, is shown. (Reproduced with permission from Davidson RJ, Pizzigalli D, Nitschke JB, Putnam K. Depression: perspectives from affective neuroscience. Annu Rev Psychol. 2002;53:545–574.)


Appraisal of a stimulus (eg, as dangerous, novel, or desirable) requires that highly processed sensory and cognitive information from the association cortex gain access to emotion processing circuits, which then activate adaptive responses. These downstream responses include stimulation of arousal and attention, mediated via activation of monoamine, cholinergic, and other widely distributed neurotransmitter systems in the brain (Chapter 6) as well as by activation of the autonomic nervous system (Chapter 9) and certain neuroendocrine systems (Chapter 10). Strong emotion suppresses ongoing behaviors in favor of automatic defensive or approach behaviors. Experiences encoded under the influence of strong emotion also lead to the enhancement of diverse types of memory processes to make subsequent responses to similar stimuli maximally efficient (Chapter 14). Whether in avoidance of a predator or in hunting food, a few hundred milliseconds can have enormous implications for success and even survival.
Research on fear in animal models has relevance to humans in both health and disease because of the evolutionary conservation of significant aspects of fear circuitry across mammalian species. Fear can be roughly divided into innate fear and learned (or conditioned) fear. Innate fear is exemplified by the inborn avoidance of brightly lit, open spaces by rodents. Conditioned fear results when an animal learns to associate predictive stimuli with danger, harm, or pain. Fear conditioning has been exploited in the laboratory to delineate fear circuitry in the brain 15–1.
15–1 Studying Fear Circuitry
Classical conditioning experiments have been used to analyze fear circuitry. In such experiments, a neutral stimulus such as a tone or light is consistently presented prior to a strongly aversive stimulus—the unconditioned stimulus (US)—such as foot shock. After a period of learning, known as conditioning, the animal regards the previously neutral stimulus, now the conditioned stimulus (CS), as a predictor of the aversive event. The resulting associative learning causes a tone previously paired with foot shock to produce fear–related behaviors, the conditioned response (CR), such as freezing or enhanced startle. (With a strongly aversive stimulus, conditioning can be apparent after a single episode.) With the use of precise lesions and anatomic methods, investigators have been able to trace the pathways between areas in the brain that receive auditory input (the tone) and the areas responsible for various physiologic and behavioral outputs (see 15–2). Such mapping studies have identified the amygdala, a complex nucleus in the temporal lobes, as a critical node in fear-processing circuits.
Corroboration of the importance of the amygdala in fear comes from observation of humans with lesions due to disease or injury and by functional neuroimaging studies following presentation of fearful stimuli. For example, the figure shows a coronal section of a functional MRI (fMRI) image. The right amygdala is significantly activated by a masked fearful face compared with a masked happy face. The rapid masking of the fearful face interferes with conscious recollection of seeing the fearful face; hence, the amygdala activation represents a rapid unconscious response. The bar graph shows the percent change in blood oxygen level–dependent (BOLD) fMRI signal for successive epochs in which individuals were exposed to masked fearful versus masked happy faces. Note that the fear response (signal elevation in amygdala) desensitizes over time.
Experiments in rat and mouse models have indicated that information about threatening stimuli is transmitted from the sensory thalamus and sensory and association areas of the cerebral cortex to the lateral nuclear complex of the amygdala (there are some species-specific differences in the organization of the amygdala), the amygdala’s major input nuclei. Information is processed and sent by both direct and indirect routes to the central nucleus, the major output nucleus of the amygdala, although important information bypasses the central nucleus and is transmitted directly to effector sites of the fear response. Efferent projections from the lateral and central amygdala activate numerous effector sites for cognitive, physiologic, and behavioral aspects of the fear response 15–2.
15–2
Flow of information into and out of the amygdala. Fear-related information enters the amygdala by means of its lateral nuclear complex. These nuclei project to the central nucleus, which projects to structures that produce the diverse physiologic and behavioral responses characteristic of fear, including the central or periaqueductal gray area, lateral hypothalamus, paraventricular nucleus (PVN) of the hypothalamus, monoaminergic nuclei such as locus ceruleus, raphe nuclei, ventral tegmental area, and many regions not shown. Several major outputs bypass the central nucleus and mediate crucial aspects of fear responses.

Projections to the lateral hypothalamus mediate activation of the sympathetic nervous system, and projections to the paraventricular nucleus (PVN) of the hypothalamus induce the synthesis and release of corticotropin-releasing factor (CRF) (Chapters 7 and 10). The release of CRF activates a cascade that ultimately leads to the release of glucocorticoids from the adrenal cortex. Glucocorticoids cause the body to enter a catabolic state; they suppress inflammatory responses and heighten arousal. The hypothalamic–pituitary–adrenal (HPA) axis often becomes chronically hyperactive in individuals with depression, as discussed in subsequent sections of this chapter. Because CRF also serves as a neurotransmitter for neurons in the central nucleus of the amygdala, through which it may contribute to fear and anxiety, CRF antagonists were considered as potential antidepressants and anxiolytics, although clinical trials have been disappointing.
Projections from the amygdala to the periaqueductal gray matter (PAG) in the core of the brainstem activate descending analgesic responses that involve endogenous opioid peptides, which can suppress pain in response to intense fear and stress (Chapter 11). Projections to a different region of the PAG activate species-specific defensive responses, such as behavioral freezing in rats and mice. Projections to the noradrenergic locus ceruleus, the serotonergic raphe nuclei, and the dopaminergic ventral tegmental area (VTA) increase arousal and vigilance and enhance the formation of explicit and implicit memories of circumstances under which danger has occurred (Chapter 6).
Under normal circumstances, fear responses enhance survival. It has been hypothesized, however, that abnormal fear processing gives rise to disabling anxiety disorders.
Anxiety is a state of preparation for danger, which is characterized by arousal, vigilance, physiologic preparedness, and, in humans, negative subjective states that are qualitatively similar to those associated with fear. Yet anxiety differs from fear in that it is triggered in the absence of an immediately threatening stimulus. Anxiety can be elicited by diverse cues ranging from the specific to the general. Moreover, in circumstances in which there are no “safety” signals, the state of anxiety may be prolonged.
The circuitry underlying anxiety may differ from that of fear responses in that the bed nucleus of the stria terminalis (BNST) may partly take the place of the central nucleus of the amygdala in giving rise to projections involved in physiologic and behavioral expressions of anxiety. The BNST is considered an extended region of the amygdala, and indeed resembles the central nucleus of the amygdala with regard to its cellular organization; yet it is hypothesized that this area may respond to less specific stimuli than the central nucleus and thus may mediate the more generalized symptoms of anxiety. CRF may act in this region to induce anxiety-like behaviors. Examples of animal tests of anxiety-like behavior are shown in 15–2.
15–2 Animal Tests of Anxiety-Like Behavior
A long-established device for observing anxiety-like behavior in rodents is the elevated plus maze (see figure). This apparatus is elevated above a table and consists of two arms that intersect to form the shape of a plus sign. The sides of one arm are closed, and the sides of the other arm are open. An animal is placed at the intersection of the arms, and the amount of time it chooses to spend in each arm is measured. Rodents normally prefer the closed arm; this preference has led investigators to hypothesize that rodents experience an aversive state akin to anxiety in the open arm. Other tests of anxiety-like behavior, such as the open field test or light–dark test, are based on similar principles, where rodents tend to avoid brightly lighted, open areas. The tests are used to screen for drugs with anxiolytic properties. Benzodiazepines such as diazepam dramatically increase the amount of time an animal spends in open areas, as indicated on the hypothetical bar graph. In contrast, β-carboline (β-CCE), an inverse agonist at the benzodiazepine binding site of the GABAA receptor, which is anxiogenic in humans, reduces the amount of time an animal spends in open areas. Whether these tests truly measure anxiety, and whether they can accurately detect anxiolytic agents of novel mechanisms of action, remains unknown.
The memories produced by fearful situations have both a cognitive and an emotional component. The cognitive component, which depends at least in part on the hippocampus, records the precise setting in which danger was experienced and details of the experience. The emotional component of fear-related memories, which depends in part on the lateral nuclear complex of the amygdala, activates physiologic and behavioral responses to danger when learned predictors of danger are encountered. Normally, we experience the cognitive and emotional components of fear-related memories as completely intertwined, but their dependence on different circuits can be revealed by lesions. Patients who have had lesions of the amygdala from stroke, viral infections, or head injury lack autonomic and other conditioned responses to previously learned fearful stimuli, even though their declarative memory (their ability to consciously recall a specific stimulus) is intact. Conversely, patients with hippocampal lesions may not consciously recall exposure to fear-associated stimuli, but retain their autonomic responses.
The cognitive component of memories encoded under strong emotion is markedly enhanced compared with ordinary memories. Contrast, for example, the memories of Americans for the details of where they were on the morning of September 11, 2001 versus September 10, 2001. Based on pharmacologic experiments in human subjects and invasive experiments in rodents, this enhancement has been shown to depend on the brain’s monoamine systems. For example, this enhancement can be blocked by β- or α1-adrenergic receptor antagonists such as propranolol and prazosin, respectively, administered systemically or directly into the amygdala prior to certain stages of memory consolidation. Evidence is suggestive that such adrenergic antagonists might be efficacious under realistic circumstances in humans, although the results are not yet conclusive. It is important that these drugs do not cause amnesia; the hoped-for goal is to cause traumatic memories to recede to the level of ordinary memories, perhaps decreasing the risk and severity of posttraumatic stress disorder (PTSD; 15–3).
15–3 Posttraumatic Stress Disorder
PTSD initially was identified in veterans exposed to life-threatening combat situations, and has more recently been demonstrated in response to severe civilian trauma. Symptoms include hyperarousal, sleep disturbance, cue-induced reexperiencing of the traumatic event accompanied by physiologic aspects of the fear response, and emotional numbing. Fear-related memories enable us to avoid putting ourselves in harm’s way a second time after we have been burned, bitten, or chased. Such memories enable us to identify cues that predict danger so that when we encounter them, we are physiologically and behaviorally ready to fight or flee. In PTSD, these memories are too strong and overly generalized and can lead to severe impairment in an individual’s ability to function.
The strength and persistence of memories encoded in the presence of intense fear may underlie not only PTSD but also other anxiety disorders, such as agoraphobia. In PTSD, reminders of the original trauma elicit both explicit (conscious) memories of the experience and emotional memories, including physiologic and behavioral fear responses. Agoraphobia, or a fear of public places, often results from the association of extremely frightening symptoms known as a panic attack with previously neutral contexts in which panic attacks have occurred. PTSD and related syndromes have been hypothesized to involve powerful stimulation of amygdala-based fear pathways, resulting in powerful, maladaptive associative emotional memories.
Positive emotional experiences also promote memory. Under normal circumstances these memories produce reinforcement in response to natural rewards such as food, safety, and mating opportunities. Just as abnormal function of fear circuitry can produce disabling symptoms, abnormal stimulation of reward circuitry by certain drugs may be a central cause of addiction. Interestingly, the amygdala, acting in concert with the brain’s reward circuitry, has been implicated in mediating such reward-enhanced memory formation (Chapter 16).
Compared with the neural mechanisms that underlie fear, those that underlie mood are less well understood, in large part due to the greater difficulty in modeling mood in animals. Experimental models of fear and anxiety in animals do not have obvious counterparts that might facilitate the investigation of moods such as sadness or happiness. Yet considerable overlap or interaction likely exists among the neural systems that regulate emotions such as fear and anxiety, and moods such as sadness. Not only do moods and emotions influence each other, but anxiety and mood disorders also often co-occur in the same individuals and appear to have shared genetic risk factors. Because of the lack of fully convincing animal models of mood, much of the investigation of mood regulation has required the use of noninvasive neuroimaging techniques such as positron emission tomography (PET) and functional magnetic resonance imaging (fMRI) in studies of human subjects. Accumulated data suggest that circuits involved in mood regulation and mood disorders include the orbital and medial prefrontal cortex, the cingulate gyrus, amygdala, ventral striatum, hippocampus, and hypothalamus.
PET studies focused attention on the region of the anterior cingulate gyrus that bends under the genu of the corpus callosum (subgenual cingulate cortex), also known as Brodmann area 25 based on traditional mapping of the cerebral cortex 15–1 and 15–3. One subregion of the anterior cingulate gyrus is activated by pain. Others are activated when task performance deviates from successful attainment of goals. Thus, the anterior cingulate cortex may monitor performance for signs of failure, just as the amygdala monitors sensory inputs for signs of threat. This cortical region exhibits, on average, increased levels of metabolic activity in major depression, which return to normal with successful pharmacologic treatment. It is also activated in normal subjects in response to scripts that induce sadness 15–3. Based on these findings, patients with unremitting major depression, who have not achieved relief from multiple drugs, psychotherapy, or electroconvulsive therapy (ECT), have been treated by deep brain stimulation (DBS) with placement of the stimulating electrode within the subgenual cingulate cortex 15–4. Such DBS might work by quieting an overactive region. These trials of DBS corroborate imaging findings in suggesting that the subgenual cingulate cortex is a critical node in mood-regulating circuits in the brain. Related studies have suggested that DBS of another site in brain—the anterior limb of the internal capsule, which includes the nucleus accumbens, a major brain reward region—may also have mood-elevating effects. These studies have prompted investigation of still additional regions of the brain that might be targeted for DBS treatment of depression. One possibility, now under investigation, is the lateral habenula. This region, which sits near the dorsal thalamus, is believed to induce aversive emotional responses via glutamatergic innervation of GABAergic neurons in the VTA, which then suppress VTA dopamine neurons.
15–3
Subgenual cingulate cortex responds to changes in mood state. PET scanning following injection of fluorine 18–labeled deoxyglucose or 15-O-labelled water permits measurement of metabolic activity or blood flow in the brain, respectively. The left panel shows patients after successful pharmacologic treatment of depression. The right panel shows normal subjects following transient induction of sadness. The top views are coronal sections; the bottom views show midsagittal sections. In the pseudocolor scale (right) red shows increased activity and blue shows decreased activity compared with the comparison state (depressed for the patients; prior to inducing sadness for the normal subjects). Successful treatment of depression decreases activity (metabolism) in subgenual cingulate cortex (cg25 or Brodmann area 25 of anterior cingulate gyrus, see 15–2), while induction of sadness increases activity (blood flow) in this area. F9 is an area in dorsolateral prefrontal cortex. Cg31 is an area in the posterior cingulate gyrus. (Used with permission from Helen Mayberg, MD.)

15–4
Deep brain stimulation for major depression. Top panel (from left to right): Stereotactic frame used in surgery; coronal section showing subgenual cingulate cortex (cg25) target; placement of bilateral electrodes, and placement of stimulator in the chest (similar to a pacemaker). Bottom panel: Activity in cg25 is elevated in a depressed patient (with elevated 17-item Hamilton depression rating scale) as measured by PET scanning following administration of fluorine 18–labeled deoxyglucose; MRI scan showing electrode placement; activity in cg25 diminishes, correlating with improvement in the Hamilton depression rating scale, measured 3 months after beginning DBS. (Reproduced with permission from Mayberg HS, et al. Deep brain stimulation for treatment-resistant depression. Neuron. 2005;45;651–660.)

Anxiety disorders refer to a heterogeneous group of conditions, each of which features some type of anxiety as a prominent symptom 15–1. The current categorization of these disorders is based exclusively on syndromal groupings of subjective and behavioral symptoms and may not reflect underlying genetic and neurobiologic features. Thus, the classification should be considered provisional and should not guide all scientific investigation. Family and twin studies suggest a genetic component of risk for these disorders. Nonetheless, the lack of objective markers for phenotyping and the likely genetic complexity of all common behavioral disorders (involvement of numerous genetic and nongenetic factors of small effect) have, to date, impeded the discovery of bona fide risk genes of strong effect. Neuroimaging studies using PET or fMRI have demonstrated hyperactive responses of the amygdala in humans with PTSD, both in response to scripts recalling their trauma and in response to more generalized stimuli such as fearful faces. Overall it appears that patients with certain anxiety disorders exhibit hyperresponsiveness of amygdala-based fear circuitry and decreased activity of medial and orbital prefrontal cortical regions that normally serve to suppress fear and anxiety. However, greater specificity is needed in delineating the neural circuits underlying the range of anxiety symptoms and disorders. Several neurotransmitter systems have been implicated in these abnormal amygdala responses, in both humans and animal models, although more research is needed to establish the cellular and molecular pathophysiology of anxiety disorders.
Panic disorder |
Panic attacks are discrete episodes of intense anxiety accompanied by somatic symptoms such as tachycardia, tachypnea, and dizziness. Panic disorder is diagnosed when multiple panic attacks occur or when one or a few attacks are followed by persistent fear of having another attack |
Generalized anxiety disorder (GAD) |
GAD is characterized by unrealistic and excessive worry for more than 6 months accompanied by specific anxiety-related symptoms, such as motor tension, sympathetic hyperactivity, and excessive vigilance |
Posttraumatic stress disorder (PTSD) |
PTSD occurs after serious trauma and is characterized by numbing, cue- elicited reliving of the traumatic experience, increased startle, and nightmares |
Simple phobias |
Simple phobias are characterized by intense fear of and avoidance of specific stimuli, such as snakes, dogs, airplane travel, or exposure to heights |
Social phobia (social anxiety disorder) |
Social phobia is a persistent fear of one or more social situations involving possible exposure to scrutiny by others, and associated fear of humiliation. An example of a specific social phobia is stage fright. A social phobia may generalize, in which case it may lead to avoidance of all social situations, resulting in substantial social and occupational disability. The boundary between shyness and this type of social phobia is not well clarified |
Despite gaps in our understanding of the genetics and pathophysiology of anxiety disorders, several highly effective treatments are available. Acute anxiety, for example, is successfully treated with benzodiazepines, generalized anxiety disorder (GAD) with antidepressant drugs or benzodiazepines, panic disorder with long-term administration of antidepressants or with high-potency benzodiazepines (often in combination with cognitive–behavioral therapies), and obsessive–compulsive disorder (OCD) with long-term administration of selective serotonin reuptake inhibitor (SSRI) antidepressants or the serotonin reuptake inhibitor tricyclic antidepressant clomipramine together with behavioral therapies. Treatment of PTSD remains challenging, although SSRIs and perhaps other antidepressants provide modest relief. For some patients, cognitive–behavioral therapies are highly effective adjuncts or alternatives to medication therapy; the efficacy of these therapies emphasizes the importance of learning and memory in the pathogenesis of many anxiety disorders. Although neurobiologic causes of anxiety are not well understood, much is known about the mechanisms by which pharmacologic agents act to reduce anxiety. Therefore, the discussion that follows approaches the topic of anxiety disorders from a treatment perspective.
As discussed in Chapter 5, the GABAA receptor, like many other ligand-gated channels, is a heteropentamer with its subunits arranged like staves around a barrel. Four major types of GABAA receptor subunits—α, β, γ, and ρ—are known, and multiple subtypes have been identified. GABAA receptors containing ρ subunits are expressed in the retina and sometimes referred to as GABAC receptors. They play no role in neuropsychopharmacology and will not be discussed further. Significant binding sites on the GABAA receptor complex are summarized in 15–2. Benzodiazepines bind to a site on the α subunit of the GABAA receptor complex and thereby increase the affinity of the β subunit for GABA; a γ subunit must be present in the heteropentamer for benzodiazepine binding and must therefore allosterically regulate the binding site on the α subunit. Benzodiazepines facilitate the ability of GABA to activate the GABAA receptor’s intrinsic Cl− channel and in turn facilitate inhibitory neurotransmission. In the absence of GABA, benzodiazepines exert little effect on GABAA receptors.
Site | Agonists | Antagonists | Inverse Agonists |
---|---|---|---|
GABA (GABAA β subunit) |
| Bicuculline | |
Benzodiazepine (GABAA α subunit) |
| Flumazenil |
|
Barbiturate |
| ||
Convulsant1 |
| ||
Neuroactive steroid2 (site uncertain) |
| DHEA-S |
Competitive antagonists of anxiolytic benzodiazepines have been discovered and include agents such as flumazenil. These antagonists do not exert independent effects on GABAA receptor function, but competitively block effects on the receptor produced by benzodiazepine agonists such as diazepam. Clinically, flumazenil is useful in the treatment of benzodiazepine overdoses; however, this drug can precipitate withdrawal in benzodiazepine-dependent patients in much the same way that opiate antagonists such as naloxone produce withdrawal symptoms in opiate-dependent patients (Chapter 16).
Inverse agonists, which decrease GABA-activated Cl− conductance, bind at or near the benzodiazepine binding site on the GABAA receptor α subunit but exert opposite effects on receptor function compared with those produced by benzodiazepines (see 15–2). The prototypical inverse agonist is β-carboline-3-carboxylic acid ethyl ester (β-CCE or β-carboline). β-CCE is proconvulsant. It is also proconflict when administered to animals undergoing the conflict test. During this test, which is used to screen for benzodiazepine effects in animals, a rat presses a lever for food or water and simultaneously receives a shock, which tends to inhibit further pressing of the lever. The pairing of these events is believed to create a state of conflict in the animal, which is faced with both the desire for food and the expectation of punishment. Benzodiazepines are anticonflict: they release behaviors inhibited by conflict. Thus, under the influence of a benzodiazepine, the rat continues to eat or drink despite the threat of punishment. β-CCE exerts the opposite effect in this assay. In nonhuman primates, β-CCE produces dose-related behavioral agitation and increases plasma cortisol, blood pressure, and heart rate. In human volunteers, inverse agonists produce sympathetic arousal and intense feelings of inner tension and impending doom.
Benzodiazepines are a class of drugs with anxiolytic, sedative, muscle relaxant, and anticonvulsant properties 15–3. Benzodiazepines also produce anterograde amnesia, a property that is exploited when high-potency, short-acting benzodiazepines are used as preoperative anxiolytics, but that otherwise represents an unwanted side effect. All benzodiazepines exert their pharmacologic effects by facilitating GABAA receptor function, as previously mentioned. A series of chemically distinct (nonbenzodiazepine) compounds, such as zolpidem, eszopiclone, or zaleplon, bind to the same site on the GABAA receptor and exert similar pharmacologic effects. Many benzodiazepines and other drugs that bind to the benzodiazepine site are available for clinical use; the major distinguishing features of these agents are their pharmacokinetic properties. Some of these drugs exhibit particularly short half-lives, and thus are desirable for use as hypnotics or sleeping pills; examples include zolpidem, eszopiclone, and the benzodiazepine lorazepam. Drugs with longer half-lives are preferable for generalized anxiety; examples include diazepam and chlordiazepoxide. Alprazolam and clonazepam are widely prescribed for the treatment of panic disorder because of their high potency. Benzodiazepines also differ based on their affinity for different GABAA α subunits, as will be discussed below. The chemical structures of representative benzodiazepines used in the treatment of anxiety disorders appear in 15–5; the structures of related agents that are more commonly used to treat insomnia are provided in Chapter 13.
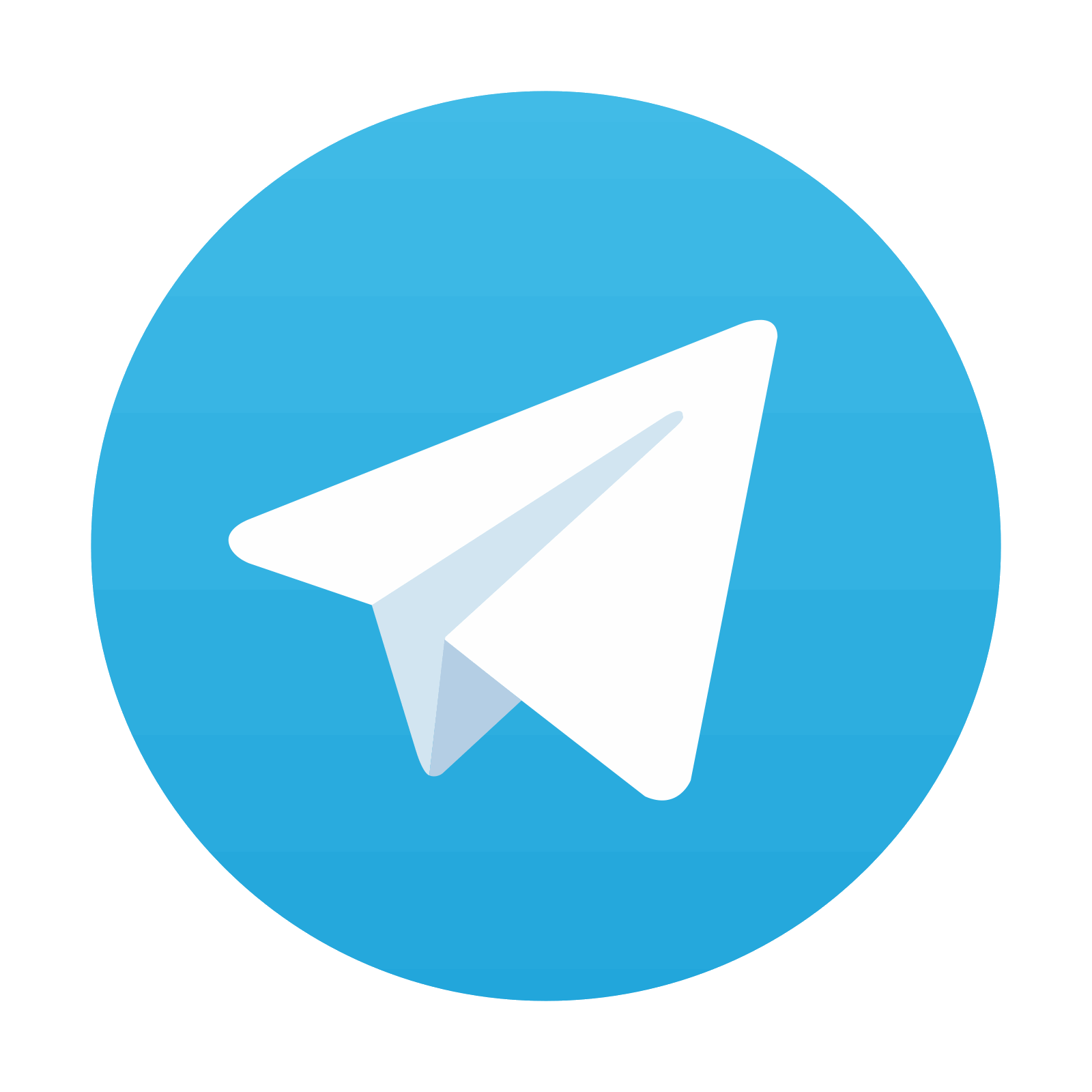
Stay updated, free articles. Join our Telegram channel
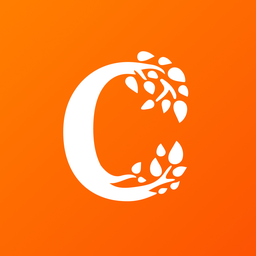
Full access? Get Clinical Tree
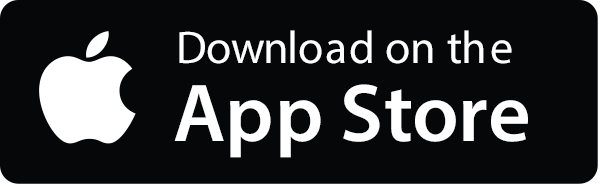
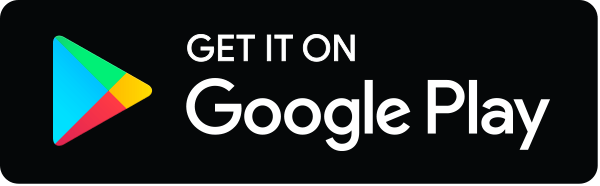