Abstract
Advances in magnetic resonance imaging (MRI) are revolutionizing our understanding of brain structure and function. The integration of this technology in patients with neuromodulation devices raises some compatibility and safety concerns. With better understanding of these interactions, investigators have successfully used functional neuroimaging to discover the mechanisms underlying therapeutic neuromodulation. Diffusion tensor imaging has improved the visualization of neural networks of interest for stereotactic targeting. More recently the integration of MRI with focused ultrasound allows for precise transcranial therapeutic ablation for the treatment of neurological and psychiatric disorders.
Keywords
Diffusion tensor imaging, Functional MRI, Functional neuroimaging, Mechanisms, Neuromodulation, Stereotactic targeting
Outline
Introduction 121
Why Do Patients With Implantable Neuromodulation Devices Need MRI? 122
MRI as a Diagnostic Tool in Functional Neurosurgery 122
Leveraging of MRI-Based Diagnostics for Advancing Neuromodulation: The Role of Functional Neuroimaging 123
MRI-Based Therapeutics: Focused Ultrasound Ablation for Neurological Disorders 124
Conclusion 125
References 125
Further Reading 127
Introduction
From the first reported applications in pain ( ), the indications for neuromodulation have broadened over the years to movement disorders, epilepsy, spasticity, and psychiatric disorders ( ). Neurostimulation systems consist of metallic electrode contacts (either paddle or cylindrical electrodes) connected to an implantable pulse generator (IPG) via subcutaneous insulated wires. The IPG is usually implanted in a subcutaneous or subfascial pocket away from the stimulation site. Various systems with this configuration are now routinely implanted for deep brain stimulation [DBS; US Food and Drug Administration (FDA) approved for essential tremor, Parkinson disease (PD), dystonia, obsessive–compulsive disorder; ], peripheral nerve field stimulation (for chronic pain and migraine; ), vagus nerve stimulation (VNS; for intractable epilepsy; ), and spinal cord stimulation (SCS; postlaminectomy syndrome and complex regional pain syndrome; ). The number of patients with implanted neurostimulation systems has steadily increased; e.g., approximately 140,000 patients have received deep brain stimulators worldwide ( http://professional.medtronic.com/pt/neuro/dbs-md/prod/- .WGQ4k4c8BHi ). SCS implantations significantly outnumber deep brain stimulators, with some estimates suggesting nearly 14,000 new implants every year around the world ( ). This increase in the number of patients with implanted neurostimulation devices parallels the larger availability and need for magnetic resonance imaging (MRI) ( ). MRI has also been leveraged to understand the mechanisms underlying the efficacy of neuromodulation systems and more recently to guide therapy. Therefore, it is imperative that clinicians in the field of neuromodulation understand the interactions between the implanted neuromodulation devices and the magnetic field during MRI, especially at higher strengths (e.g., 3 T). Potential interactions include the effects on imaging processes, such as distortions, that affect the image quality (e.g., an in situ neurostimulator device can induce susceptibility artifacts at the tissue–electrode interface), but more importantly, the potential loss of efficacy and even thermal injury to patients. Safety concerns have led a number of centers to avoid performing MRI in patients with indwelling neurostimulators. In a survey of 42 National Parkinson Foundation Centers of Excellence, 17 were not performing postoperative MRI in DBS patients, mainly to adhere to industry-proposed warnings ( ). Sometimes patients are faced with the proposition of explanting their neurostimulator to obtain a diagnostic MRI study ( ). Therefore it is important to critically evaluate the need for MRI in patients with implanted neurostimulation systems, understand the safety concerns, and monitor critical safety parameters. The safety of MRI in patients with implanted neurostimulators is discussed elsewhere in this book. Here we briefly discuss the current indications of diagnostic MRI in this population, and review in detail the role of MRI, particularly functional neuroimaging, in advancing the field of neuromodulation, both for improved diagnostic studies and for MRI-guided therapy.
Why Do Patients With Implantable Neuromodulation Devices Need MRI?
MRI is preferred to computed tomography (CT) scans because of the lack of ionizing radiation and the range of sequences available for investigating anatomy and function, which include structural anatomy, blood flow, spectroscopy, functional connectivity, structural connectivity, etc. There are at least four different scenarios in which patients with implanted neurostimulators require MRI. A majority require it as a part of the diagnostic workup for various conditions such as acute ischemic stroke with diffusion-weighted imaging ( ). estimated that nearly 82% to 84% of patients with an implanted spinal cord stimulator will require an MRI within 5 years of implantation, mainly for nonspinal indications. MRI is the modality of choice for conditions such as osteoarthritis and suspected stroke, and the American College of Radiology classifies these as the “criteria for diagnostic MRI with no equivalent test holding a similar rating” ( , https://acsearch.acr.org/list ). The second scenario involves postoperative evaluation to document electrode location and potential surgical complications such as postoperative stroke ( ). The superior anatomic definition enabled by MRI compared to CT imaging has progressively shifted the clinical work flow in favor of MRI use ( ). Third, intraoperative MRI guidance is increasingly being used for stereotactic surgery because of its superior image resolution ( ). Finally, for research applications, MRI is the modality of choice, especially in this era of “connectomic surgery” for emerging neuromodulation indications such as mood and cognition disorders ( ). Important insights into connectivity-based models of brain function ( ) have transformed our understanding of mechanisms underlying the therapeutic efficacy of DBS and SCS ( ). This framework will be critical for testing therapeutic neuromodulation hypotheses in the future ( ).
MRI as a Diagnostic Tool in Functional Neurosurgery
DBS conventionally involves the stereotactic implantation of a quadripolar electrode in a subcortical nucleus. The accuracy of implantation, defined as the difference between the intended (or planned) and the actual (or implanted) implant coordinates, is an important determinant of outcomes ( ). Surgeons recognize that, among other factors, the degree of brain shift ( ) pneumocephalus ( ), deformation of the electrodes ( ), and sequence of electrode implantation ( ) affect implantation accuracy. Overall the accurate identification of the target nucleus is fundamental to achieving a good clinical outcome. As of this writing, stereotactic targeting during DBS surgery is performed with atlas-based or formulaic methods using structural imaging and intraoperative confirmation with microelectrode recordings ( ). This targeting approach does not sufficiently incorporate individual anatomical and functional variations ( ). In addition the risks associated with multiple microelectrode tracks ( ) and excess operating times for target refinements add to the overall risks and costs. Several imaging sequences have provided better visualization of deep gray nuclei for targeting. For example, the 1.5-T susceptibility-weighted imaging MRI sequence provides better contrast of subthalamic nucleus (STN) boundaries than the T2 sequence at 1.5- and 3-T MRI ( ). However, 1.5-T MRI consistently underestimates the anterior and lateral STN boundary, compared with microelectrode recordings. Similarly the 3-T fast gray matter acquisition T1 inversion recovery sequence has improved visualization of the globus pallidus and the STN ( ). This sequence provides good imaging resolution of boundaries, but has not been compared with microelectrode recording ( ).
There is emerging evidence that stimulation of white matter tracts surrounding the stimulation target may be partly responsible for the therapeutic efficacy of DBS ( ). analyzed white matter tracts associated with good clinical outcome in 22 patients after bilateral STN DBS. The authors calculated the volume of tissue activation models from active contact settings and used it as a seed for tractography. Tractography was accomplished using an integrated processor called DBSproc that aims to provide an “all-in-one” solution for contact reconstruction from CT images to actual “realistic” diffusion tensor imaging (DTI), based on the volume of tissue activated (VTA). A probabilistic tractography algorithm was used from the FATCAT suite ( ). The authors were able to show that clinical effectiveness was positively associated with strong connectivity to the superior frontal gyrus and the thalamus. performed a probabilistic high-angular-resolution diffusion imaging–based tractography and resting state functional MRI (fMRI) analysis in 12 healthy volunteers. Their findings suggest the “hyperdirect” pathway, from motor STN to motor cortex, as a substrate for DBS efficacy in PD. Similar investigations have been performed in patients with dystonia and implanted bilateral pallidal DBS ( ). In tremor, the dentatorubrothalamic tract is thought to be responsible for DBS-mediated tremor suppression ( ). The clinical application of DTI is limited by concerns regarding accuracy ( ). Therefore the incorporation of tractography for stereotactic targeting requires the development of consistent frameworks for imaging acquisition, preprocessing, and tract visualization ( ). Deterministic tractography is the technique of choice for tractography-based targeting for stereotactic surgery ( ). With this goal in mind a tractography-based ventral intermediate nucleus (VIM) targeting methodology was developed for tremor surgery ( ). The VIM was visualized based on the relative location of the neighboring pyramidal tract and medial lemniscus. The tractography-based VIM location correlated well with the physiology-based location for VIM ( ). The initial clinical results using this methodology are promising and investigations are under way to explore long-term outcomes and compare it with conventional targeting methods.
Leveraging of MRI-Based Diagnostics for Advancing Neuromodulation: The Role of Functional Neuroimaging
DBS is a transformative neuromodulation therapy for many patients with PD and essential tremor. However, achieving consistent clinical outcomes remains a major focus of research addressing this challenge. Functional neuroimaging is vital for this research by enhancing the understanding of the mechanisms underlying DBS efficacy, precise identification of therapeutic zones in the brain, and selection of stimulation parameters for the most efficacious network modulation. fMRI in particular is an invaluable tool to assess network dynamics modulated by neurostimulation and therefore reveal the key mechanisms underlying DBS or SCS efficacy.
fMRI is based on measurement of the blood oxygen level-dependent (BOLD) metabolic signal and has correlation with neurophysiological measurements ( ). fMRI enables monitoring of brain networks, which include distant but functionally connected regions, underlying the intrinsic mechanisms of brain function. In contrast to fMRI, DTI and probabilistic tractography estimate the direct structural connectivity between two brain regions by using complex mathematical modeling of the diffusion of water molecules ( ). These complementary modalities provide a unique opportunity to noninvasively study brain connectivity (functional connectivity with fMRI and structural connectivity with probabilistic tractography) and therefore have proven to be major drivers of growth in the neurosciences ( ).
In the field of neuromodulation, fMRI is becoming the modality of choice with an increasing understanding of the safety of neuromodulation hardware in the MRI environment ( ). Phantom studies show a negligible rise in temperature at the electrode tip during echo-planar imaging acquisitions in both 1.5- and 3-T environments ( Fig. 11.1 ) ( ). published the first report of fMRI performed in three patients with chronic pain and three patients with essential tremor with externalized DBS leads using a 1.5-T system ( Fig. 11.2 ). The authors demonstrated congruent cortical activation at clinically effective parameters, with no reports of adverse effects from the MRI radio-frequency-field interference. In another investigation ( ), fMRI was performed in a PD patient with stimulation-induced mood changes (dysphoria) after bilateral STN DBS. In this study the right electrode was implanted in a more superficial location, far from the “effective” location in STN, and it demonstrated a different pattern of connectivity compared to the efficacious electrode. Several other investigations have been published since then confirming the earlier findings ( ). showed a stimulation-associated reversal of the effective connectivity between motor cortex and thalamus in PD with administration of DBS. Investigations have also demonstrated modulation of both motor (premotor and motor cortices, thalamus, and contralateral cerebellum) and nonmotor networks with clinically efficacious STN DBS ( ).


fMRI investigations have been undertaken with other neuromodulation devices as well. For instance, performed 1.5-T fMRI in six patients implanted with vagal nerve stimulators, which are used to treat patients with drug-resistant epilepsy and depression ( ). The authors found increased BOLD fMRI response in the orbitofrontal cortex, frontal pole, hypothalamus, left pallidum, and thalamus associated with active VNS therapy at 20 Hz. This setting also changed the BOLD response to an auditory stimulus during the scan, compared to a 5-Hz stimulation. Similar results have been reported by other groups ( ). studied the BOLD changes associated with transcutaneous VNS in 22 healthy volunteers, and found a robust transcutaneous VNS–induced BOLD signal decrease in limbic brain areas, including the amygdala, hippocampus, parahippocampal gyrus, and middle and superior temporal gyrus, but an increased activation in the insula, precentral gyrus, and thalamus. Most recently, investigated the mechanisms underlying the therapeutic efficacy of SCS using resting-state fMRI with 3-T fMRI ( Fig. 11.3 ). The authors reported that efficacious SCS decreased functional connectivity between somatosensory and limbic areas. Similar reports have been published using VNS ( ), highlighting an increasing interest in this field of research. fMRI represents a complementary approach to DTI for personalizing targeting and improving patient programming strategies after DBS surgery. The opportunity to perform postoperative high-field (3-T) MRI imaging in patients with implanted hardware ( ) will potentially allow a real-time readout of network dynamics associated with therapeutic neuromodulation. Although the use of 3-T imaging has been previously shown to be safe and feasible ( ), more investigations are required to conclusively define the safety parameters to routinely perform these studies in the future. With multimodality imaging initiatives like the Human Connectome Project, the parcellation of the human cortex is being performed with an unprecedented level of detail ( ). Incorporating this information into the field of neuromodulation will allow a deeper understanding of the mechanisms underlying therapeutic efficacy and may enable the identification of specific neuroimaging markers for therapeutic efficacy. It is critical that the clinical implementation of both of these neuroimaging modalities requires steps to minimize errors and limitations associated with imaging acquisition factors (subject motion, artifacts due to sequence, tissue inhomogeneity, voxel size) and preprocessing time. In addition, clinical implementation requires validation of results in difficult scenarios such as brain atrophy or conditions without definitive disease markers ( ).
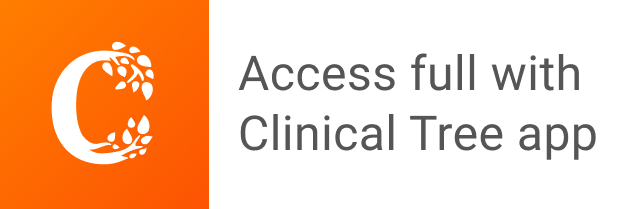