Neocortical Anatomy and Physiology
Kristen A. Richardson
Erika E. Fanselow
Barry W. Connors
Introduction
The neocortex in humans is a sheet of gray matter, 2 to 3 mm thick, with a surface area of about 2,400 cm2, the size of a chess board. Like crumpled paper, the neocortex is folded into multiple gyri and sulci, and so fits compactly into the cranium. Gyri and sulci may be more than a solution to a packaging problem; by one view, they may also serve to compartmentalize neocortical functions.192 Neocortex, together with the white matter that makes up its axonal connections, comprises about 80% of human brain volume.137 Not necessary for life itself, the neocortex is essential for proper perception, motor control, memory, and cognition. Its neural circuits can adapt to a wide variety of functions, and the behavioral characteristics that define humanity can undoubtedly be ascribed to the unique expanse of neocortex enjoyed by our species. Unfortunately, possession of a neocortex comes with significant risk. The neurons and circuits that are fundamental to its normal operations can, with little provocation, produce the spectacularly disruptive activity of a seizure.
The mechanisms of clinical seizures in the neocortex are only poorly understood. However, knowledge of the basic anatomy and physiology of the neocortex is essential even to frame the questions about seizure mechanisms. Indeed, an interest in seizures has inspired many basic studies of the neocortex. The literature on neocortical biology is appropriately immense and eclectic, and numerous reviews are available.1,25,50,57,77,125,128,129,141,194 This chapter briefly describes the structure and function of the neocortex, emphasizing those features that may contribute to its epileptic tendencies.
Basic Anatomy and Physiology of the Neocortex
The intricate structure of the neocortex has resisted explanation for at least 150 years.95 On the one hand, all neocortex—from mouse to man—shares a set of common properties: The histologic appearance is comparable, the classes of neurons and the gross pattern of their connections are very similar, and the same transmitters are in use. Nevertheless, the neocortex is subdivided into many distinct areas, perhaps 50 to 100 in humans, and the number and types of areas vary widely among species. The important distinguishing features of each area include variations in cell lamination (useful for identification of the area), the patterns of axonal connections (hinting at the function of the area), and the details of neuronal structure, local interconnections, and biochemistry and gene expression (fodder for mechanistic speculations). Areas may also differ in the physiologic properties of their neurons and synapses. Nevertheless, despite knowing a tremendous amount about the general and specific structure and function of the neocortex, it is still fair to say that we cannot describe with confidence what the fundamental task of any one area of neocortex is.
Lamination as a Structural Principle
Anatomists have settled on a general six-layer nomenclature for the neocortex, although some layers can be further subdivided in many cortical areas and species.24,104 Layering is easy to see, so the different lamination patterns of neurons, myelin, or various other structural features (glia, blood vessels, biochemical markers) have served as the basis for many schemes for neocortical parcellation. The most common approach to neocortical cytoarchitecture is Nissl staining, which highlights the cell nuclei and darkly staining clumps of material around the nuclei of neurons (Fig. 1). The Nissl-stained neocortical layers are distinguished by the density and size of their constituent neurons. In general, layer I (the “molecular” layer) is just below the pia and is thin and virtually neuron free. Layers II and III are hard to separate in many areas and contain medium-sized neurons in moderate density. Layer IV is the “granular” layer because of its densely packed, small neurons. Layer V contains the largest neurons of the neocortex and includes the output cells. Layer VI (the “multiform” layer) has a wide range of neuronal sizes and shapes. This general scheme has myriad variations that early anatomists, notably Brodmann, used to distinguish and define the areas of the neocortex. For example, Brodmann’s area 17 (primary visual cortex) has a thick layer IV with a “striated” appearance, whereas area 4 (primary motor cortex) lacks an obvious layer IV, but has exceptionally large, pyramidal-shaped neurons in layer V.
Modern anatomic methods show that the axonal connections of the neocortex are also distinctively laminated (Fig. 2). As a rule, specific neural information enters an area of the neocortex only via axons from the thalamus or other areas of cerebral cortex. Additional sources of extrinsic input include a variety of nuclei in the brainstem that project diffusely across the neocortex and deliver modulatory substances, such as norepinephrine, serotonin, acetylcholine, and dopamine.121 Thalamic afferents from specific nuclei terminate primarily within layers III, VI, and (especially) IV. However, all other cortical layers may also receive thalamic input, depending on the area and thalamic nucleus in question.81 Although thalamic axons are the source of input to the neocortex from the external world, they provide only a minority of all cortical synapses; even in layer IV, only 5% to 20% of synapses are from the thalamus.142,194 Most of the rest of neocortical synapses are from the neocortex itself. Neurons in layers II and III project densely upon layers III and IV (primarily) in other areas, whereas cells in layers V and VI project to distant areas and terminate above and below layer IV.
Vertical Organization of Neocortex
Nissl stains give the illusion that the neurons of the neocortex are like so many stratified grains of sand and gravel. Despite variable lamination, there is surprising uniformity of dimension. Across cortical areas and even across species, the number of neurons within a vertical column of gray matter with a surface area of 1 mm2 is nearly identical.148 Although some variations exist among areas,13 the only major exception to this rule of uniformity is the primary visual cortex of primates, which has twice the neuron density of most other areas.
Nissl stains illuminate only somata, but the vast majority of each neuron’s volume and surface area is in its dendrites and axons, which may stretch across layers and between areas. In the neocortex, a tendency exists for these cellular processes to extend strongly in the vertical dimension and for cells in vertical arrays to be densely interconnected. Pyramidal cells, in particular, seem to have evolved an efficient shape to collect synaptic input from a vertically oriented zone of tissue that may span several layers.56,174 Physiologic studies show that the sensory or motor response properties of neurons in vertical “columns” of neocortex are often quite similar, whereas neighboring neurons along the horizontal dimension are relatively more different.9,86,127,128 The widespread tendency for neocortical neurons to organize into vertical groups has prompted speculations that the neocortex is basically modular128; small columns form the irreducible units of neocortical organization, and new areas and functions are built up by simply adding more columnar modules. This scheme is appealing but unproven.
Systematic anatomic and physiologic measurements have suggested a basic vertical circuit for neocortex.7,8,72 It begins in layers III and IV, where the main thalamic input arrives. Local axons of the spiny stellate cells in layer IV then excite pyramidal neurons in the upper layers, which in turn strongly excite neurons of layer V. Layer V cells synapse upon cells of layer VI, and these return excitation both to the thalamus and to layer IV. The neocortex is primarily feeding information back upon itself, which may be the single most important thing we can say about its susceptibility to seizures. If excitation were the only story, however, the cortex would seize interminably. In parallel with the loops of excitatory circuitry, γ-aminobutyric acid (GABA)-releasing neurons also receive excitation and, in turn, inhibit pyramidal neurons, both ahead of and behind them in the circuit flow.42,96,162
Topologic maps of the vertical organization of neocortex have been described for many different areas and species; these reveal that, in many cases, “columns” have been misnamed. For example, in visual cortex, the zones specific for ocular dominance look, from the surface, like alternating bands or slabs, whereas the organization of neurons with similar stimulus orientation preferences resembles radiating pinwheels.11,21 Furthermore, these two maps of visual function are coextensive—they use the same sheet of cortex simultaneously but divide it quite differently. For the most part, the anatomic underpinnings of functional columns have not yet been demonstrated in detail.28,83 In a general way, vertical connectivity must link neurons across layers, but with a high degree of specificity.
Horizontal Organization of Neocortex
Although vertical connections received early114 and sustained publicity, the horizontal connections of the neocortex are also widespread, complex, and essential. Their existence provides a ready set of pathways for the rampant propagation of seizures. A few generalizations about horizontal connections can be made: They vary from very short to very long, they are reciprocal, and they tend to terminate in discontinuous, patchy patterns.
In a single brain, the neocortex consists of two continuous sheets, one in each hemisphere. Horizontal connections exist between points on this sheet at very local scales (within single microcolumns), at intermediate scales between columns, within areas, between adjacent areas, and at long distances between far-flung ipsilateral areas, as well as between homologous contralateral areas via connections through the corpus callosum. In most cases, the horizontal interconnections at all scales are reciprocal; a set of connections from cell group A to cell group B is matched by a set of connections from B to A. The patchiness of interconnections is nearly ubiquitous, although there are exceptions,185 and both the regular patterns of connections and the scale of their periodicities tend to be strikingly similar across cortical areas.73,97,110,111
Horizontal interconnections, especially over intermediate and long distances, are overwhelmingly excitatory, reinforcing their relevance for the spread of seizure activity. Experimental work has shown that local variations of horizontal excitatory connectivity are an important determinant of the speed and route of seizure propagation.35,143,183 At shorter distances, on the order of adjacent intercolumn distances within one area, there may be a liberal mix of horizontal inhibitory and excitatory connections. The spatial details and relative strengths of these connections are variable with area and are probably critical in helping to determine the unique functions of each area.
Growing evidence also suggests that horizontal connections mediate some forms of experience-dependent plasticity in sensory and motor areas of the neocortex.71,153 Cortical synapses can either strengthen or weaken, depending on their recent history of activity,12 axons and synapses remodel dynamically165 and, over weeks and months, cortical axons can also sprout new branches in response to chronic alterations of thalamic input.53 It seems very likely that the grossly abnormal activity of chronic seizures could induce significant physical and physiologic changes in cortical circuitry.
Diverse Neurons of Neocortex: Morphology
At first glance, the neurons of the neocortex are dazzling in their diversity. By examining cellular morphology, connections, biochemistry, and physiology, however, order emerges.4,195 The neurons can be divided into two major groups based on structure—spiny neurons (about 70%–85% of the total cells) and aspiny (“sparsely spiny” or “smooth”) nonpyramidal neurons.56,141,162 Spines are small (about 1–0.2 μm on average), membranous excrescences along the shafts of dendrites.
Within the cortex, spines are notable as the major site of excitatory synaptic input onto spiny cells. The spiny and aspiny morphologic categories also correspond to basic cellular functions. Spiny cells make excitatory synapses that use the excitatory amino acid glutamate; aspiny nonpyramidal neurons make inhibitory GABA-utilizing synapses. Neurotransmitters of the neocortex will not be specifically covered in this chapter, but numerous reviews are available (see Chapters 22 and 23).
The two major classes of cortical neurons, in turn, are subdivided. The excitatory, spiny cells include pyramidal cells and spiny nonpyramidal cells (often called spiny stellate cells). Pyramidal cells are the majority class of neurons in the neocortex. They have a high density of dendritic spines (rare exceptions to this rule exist), prominent apical dendrites, and an axon that projects out of the cortex, as well as locally. Pyramidal cells vary widely in size, shape, axonal structure, and their somata appear in all layers except layer I.56 Spiny stellate (nonpyramidal) cells are relatively small neurons of layer IV, with high spine density and axons that terminate in excitatory synapses. Spiny stellate cells are distinguished from pyramidal cells by the
absence of an obvious apical dendrite, and their axons usually do not leave the cortex (although a minority may send axons out of the cortex).188 Because of their excitatory function and spiny dendritic structure, spiny stellate cells may be considered a simple variant of the pyramidal cells. This view is supported by the development of spiny stellate cells; apparently, they begin life as pyramidal neurons, and during postnatal maturation, they gradually retract their apical dendrites.187
absence of an obvious apical dendrite, and their axons usually do not leave the cortex (although a minority may send axons out of the cortex).188 Because of their excitatory function and spiny dendritic structure, spiny stellate cells may be considered a simple variant of the pyramidal cells. This view is supported by the development of spiny stellate cells; apparently, they begin life as pyramidal neurons, and during postnatal maturation, they gradually retract their apical dendrites.187
The inhibitory, sparsely spiny, nonpyramidal neurons are a very diverse group.102,118,195 Whereas their dendritic patterns vary systematically,37 a more useful characteristic is the pattern of their axon arbors, which do not leave the local area of cortex but which may be distinctive and specific.61 As schematized in FIGURE 3, common morphologies for inhibitory interneurons include double bouquet cells, whose axons and dendrites project within a long, narrow, vertical column; bitufted cells, whose collaterals form two highly branched protrusions from the soma; bipolar cells, which have long collaterals projecting vertically above and below the soma; multipolar cells, which have round somata with multiple dendrites projecting radially; neurogliaform cells, whose dendrites form relatively tight, symmetrical spheres around their somas; basket cells, whose axons tend to form basket-like shapes around the somata of their target cells; Martinotti cells, which can have their soma in layers II to V and project their axon up to layer I, where they ramify up to several millimeters; and axo-axonic cells, whose dendrites terminate on the axon initial segments of target pyramidal cells, forming structures that resemble candlesticks (thus the alternative name, chandelier cells). Note that this is not an exhaustive list of inhibitory neuron morphologies, nor do neurons always fit clearly into one morphologic subtype.
It has been shown that somatic and perisomatic targeting cells can readily suppress action potentials,124 suggesting that these inhibitory neurons could closely control the output of their target neurons. Because of their potentially strong control over pyramidal cell output, loss of axo-axonic cells has been implicated in epilepsy.55 It has also been proposed, paradoxically, that axo-axonic cells actually excite pyramidal neurons because of a relatively high internal chloride ions [Cl–] in axon initial segments.173
Other inhibitory neurons terminate on the dendrites of their target cells. Examples include double bouquet cells, bitufted cells, bipolar cells, neurogliaform cells, and Martinotti cells (Fig. 3A–C, E, G). The nature of the inhibition from these cells probably differs from interneurons that synapse in somatic or perisomatic regions. Dendrite-targeting inhibitory cells can alter the way their target cell integrates synaptic input within the dendrites, and it is thought that these cells can suppress dendritic calcium ion (Ca2+) spikes,106,124 decreasing the ability of excitatory inputs to propagate along the dendrites.
Classes of inhibitory cells may also be defined by interesting variations in peptide cotransmitters (in addition to the GABA that they all contain),80 by different types of Ca2+ binding proteins they express,100,184 and by their expression patterns of a wide variety of genes.22,131,170 Three main Ca-binding proteins have been useful as markers of interneuron types: Calbindin (CB), calretinin (CR), and parvalbumin (PV). The most common neuropeptides used to identify inhibitory interneuron subtypes include cholecystokinin (CCK), somatostatin (SOM), and vasoactive intestinal peptide (VIP). Double labeling studies have shown that several of these markers tend to co-exist within cells (e.g., CR and VIP), but others never or very rarely co-exist (e.g., PV and SOM). Kawaguchi and Kubota103 divided prefrontal neocortical inhibitory neurons in layers II through VI into four main groups based on these markers. Group I contains PV; group II contains SOM and neuropeptide Y (NPY); group III contains CR, VIP, and CCK; and group IV contains large CCK cells. However, it is not clear that these groups correlate well with specific morphologic or physiologic subcategories of inhibitory neurons. One pattern that does emerge is that PV
appears to be associated with somatic/perisomatic or axo-axonic targeting cells, whereas CR is largely associated with dendritic targeting cells (reviewed in118).
appears to be associated with somatic/perisomatic or axo-axonic targeting cells, whereas CR is largely associated with dendritic targeting cells (reviewed in118).
Diverse Neurons of Neocortex: Physiology
Just as neurons vary in their shape, transmitter, and synaptic connections, they may also differ in their intrinsic physiologic properties.46,49,112 “Intrinsic physiology” is defined as a neuron’s inherent ability to respond to stimuli, apart from the effects of the neural network surrounding it; intrinsic physiologic properties derive from the cell’s membrane (and its ion channels, primarily) and metabolic properties (such as ion pumps and control systems, second-messenger systems, and energy management). Takahashi176 was the first to show that the intrinsic properties of neocortical cells had diverse properties. Recording from the pyramidal tract neurons of the cat, he found that axonal conduction velocities correlated with somatic spike duration, time constant, input resistance, and duration of the afterhyperpolarization. Since then, numerous studies have used methods of intracellular recording, single-cell staining, and isolated brain slices in vitro to demonstrate that the intrinsic physiology and morphology of neurons in the neocortex are related. The impact of a neuron’s intrinsic properties can be appreciated from its response to simple injected current stimuli, as shown in FIGURE 4. Some cells produce a tonic, uniform spike frequency; others display strong adaptation; and still others generate periodic bursts. Clearly, when faced with the same input, each of these neurons provides a very different message to its postsynaptic targets. Myriad variations on these basic themes exist in the neocortex and in the rest of the brain.
Regular-Spiking Pyramidal Cells
The “regular” intrinsic firing pattern130 (regular implying most common) is that of most pyramidal cells from layers II to VI.47,122,164 Regular-spiking (RS) cells usually fire a single spike to a threshold current pulse. Their action potentials rise at a higher rate than the rate at which they fall. These may display a combination of fast, medium, and slow afterhyperpolarizations, as well as a brief afterdepolarization.29,47,115,155
Repetitive firing has an initially high frequency that subsequently shows strong adaptation; that is, spike frequency declines during a sustained stimulus. Most RS cells adapt from a beginning rate of several hundred hertz to a relatively stable, but much lower, rate within about 200 msec. The adapted rates are rarely higher than 50 to 100 Hz. Some RS cells adapt steeply and often stop altogether, even as stimulus current is maintained.2,33 One subclass of nonbursting pyramidal cell displays no frequency adaptation but generates a very rhythmic pattern of single spikes instead.158 Near threshold, at about –65 to –60 mV, these cells can be bistable, shifting from silence to repetitive firing at low (5–12 Hz) rates when triggered by brief stimuli.
Intrinsically Bursting Pyramidal Cells
A particularly interesting type of cortical neuron, especially in the context of seizure generation, is the intrinsically bursting (IB) cell. Although RS cells generate a single spike to a just-threshold stimulus, the minimal response of the IB subgroup of pyramidal cells is usually a high-frequency cluster of action potentials or “burst.”47,109,122 Each burst consists of 3 to 5 spikes firing at about 150 to 300 Hz. Bursts occur either singly or in repeating patterns of bursts at 5 to 15 Hz.2,34,158 Near firing threshold, repetitively bursting neurons of layer V can also exhibit a bistable state similar to the single-spiking cells described in the previous section. Small triggering stimuli generate long-lasting responses consisting of rhythmic bursts or burst-single spike complexes. These IB cells have been implicated in the initiation of epileptiform activity in experimental studies of neocortex in vitro.44
Although most physiologic studies of neurons have actually been studies of their somata, this misses most of the point for pyramidal cells; as much as 97% of their membrane area is actually dendritic,109 and 1 mm3 of neocortical gray matter contains a total dendritic length of about 450 m.25 The patch-clamp method has allowed direct recordings from cortical dendrites. These have shown that the dendrites of pyramidal cells are quite excitable and express sodium (Na),88,168 Ca,5,106,116 and potassium (K)94 currents that can powerfully affect the cells’ ability to transform synaptic inputs. Indeed, electrically excitable dendrites are probably essential for the efficacy of many distal synaptic inputs onto the longest dendrites of neocortex—synapses in layer I onto the dendrites of cells with their somata in layer V, for example.31 In addition, just as the intrinsic physiology of neuronal somata can vary in neocortex, the physiology of long dendrites can also vary systematically from one cell type to another.106 The properties and significance of electrically excitable dendrites are being intensively investigated.167,191
The density of IB cells is strikingly dependent on the cortical layer. In rodent sensorimotor cortex, their somata are most frequently observed in layer V—its deeper aspects in particular.34,122 However, they are also seen in layer IV in some species and cortical areas.34,47 Most or all of the layer V IB cells are subcortical projection neurons.98,190
Diverse Physiology of Inhibitory Interneurons
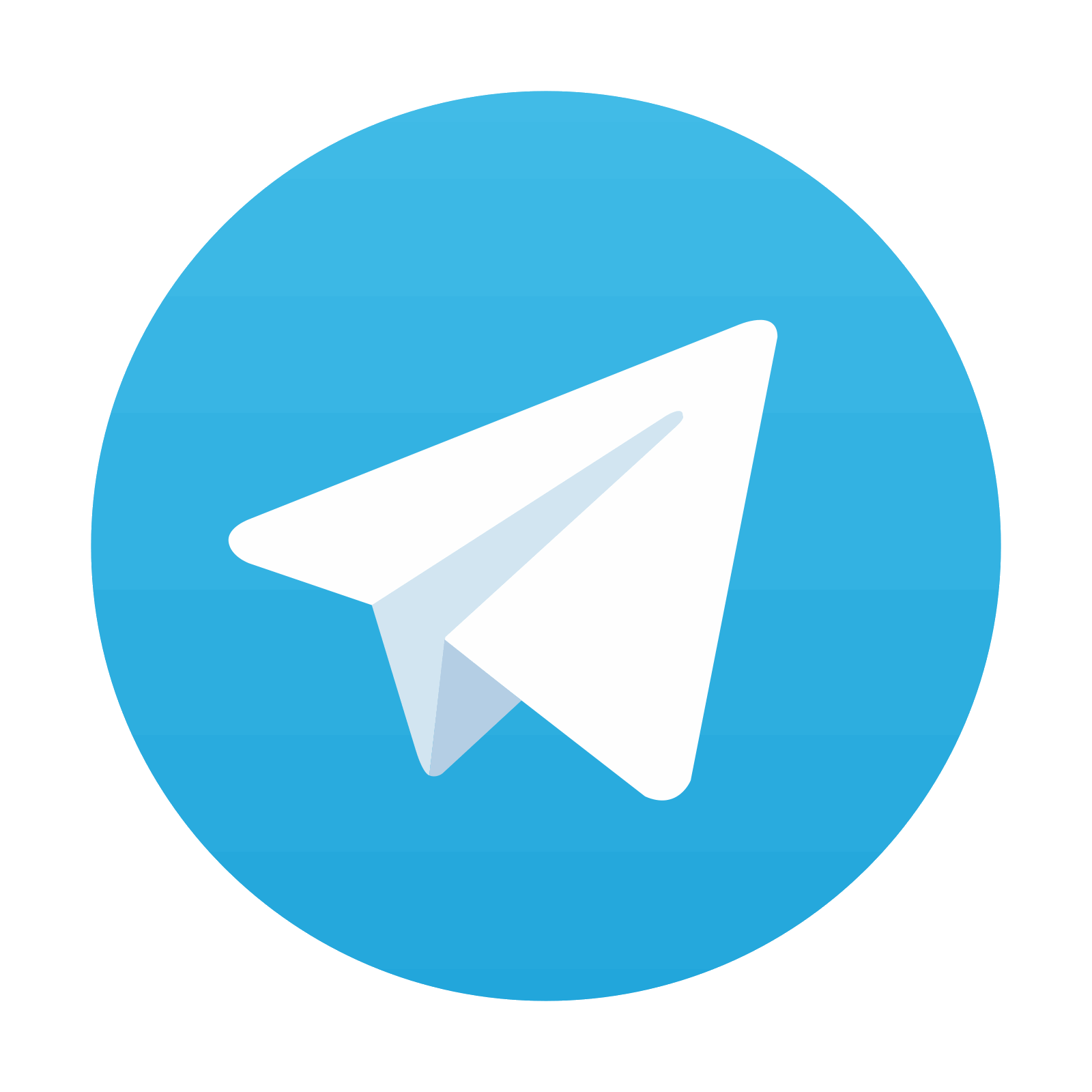
Stay updated, free articles. Join our Telegram channel
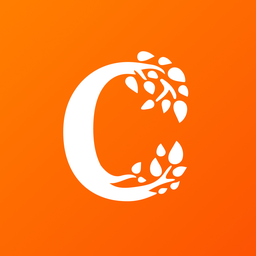
Full access? Get Clinical Tree
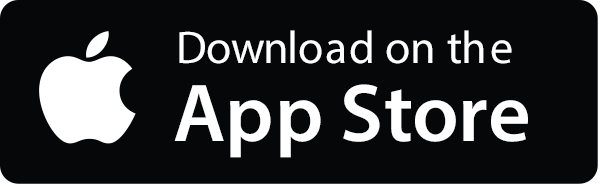
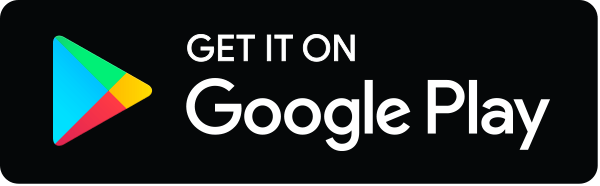
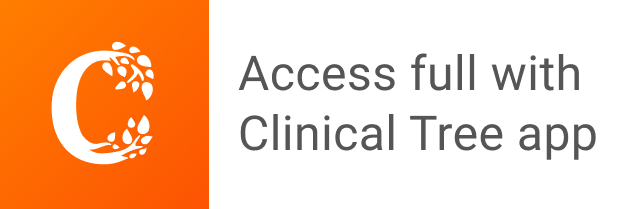