Neural Development in Invertebrates
Roger P. Croll
11.1 Overview of Invertebrate Development
Invertebrates comprise the vast majority of animal species on Earth and exhibit a diverse range of body forms which arise through different developmental programs. Furthermore, many invertebrates possess complex life cycles with larvae often differing greatly from adults in morphology, habitat, and behavior. In fact, some invertebrates have multiple sequential body forms which bear little resemblance to one another and, at first glance, may be mistaken for entirely different species. As if all this were not enough to thoroughly confuse any reader seeking a simple description of neural development in invertebrates, some of these animals can develop into identical adult forms by alternative methods (e.g., through sexual reproduction or through asexual budding).
Experience with our own species, common pets, and perhaps a vertebrate‐oriented research career might imbue many neuroscientists with a view of early animal life as a period of development sheltered by gestation and parental care from which a smaller individual, which is more‐or‐less similar to the adult, may emerge to complete its final growth in preparation for later reproductive activities. Such a perspective is misleading for understanding the development of invertebrates. While a few invertebrates may undergo transitional periods of development sequestered from many of the demands of daily life (e.g., during the pupal stages of insects), most invertebrates are free‐living throughout almost the entirety of ontogeny. These animals must grow, sometimes by enormous proportions, and undergo dramatic changes in body shape while their nervous systems continue to respond appropriately to stimuli and produce the motor programs that underlie feeding, locomotion, and escape. Furthermore, often radical transitions in body form must be accomplished quickly (e.g., within the course of a tidal cycle; Hadfield, 2000).
To understand the development of invertebrates and their nervous systems one must also jettison the idea that each developmental stage is simply a rudimentary and imperfect intermediate form in a continuous linear path toward the construction of a mature, reproductive adult. Instead, it must be remembered that each stage of the free‐living larva is exposed to selective pressures which shape its form and function. Thus larval stages can and do evolve very differently from the adult stages. In extreme cases, a completely new larval form may be inserted into a developmental plan, permitting growth and dispersal of the species without competition for resources with the reproductive adult. The literature thus refers to primary and secondary larvae, contrasting larvae representative of ancestral forms with those that evolved subsequently (Raff & Byrne, 2006). Significant debates have involved not only questions of phylogeny, but also whether comparisons are most appropriate between larval or adult stages.
A final cornerstone of this chapter is a recommendation as to the level of analysis and perspective that a reader should bring to invertebrate neural development. As mentioned in other chapters, an important driving force in such studies is the comparison of homologous gene networks. However, knowledge of genes themselves provides little insight into how the nervous system controls the physiology and behavior of a developing organism. Furthermore, in many invertebrates, our current knowledge consists only of the existence or nonexistence of certain well‐conserved genes—and perhaps their spatiotemporal expression patterns—while their contextual function remains largely unexplored. The focus of this chapter will therefore be dedicated to descriptions of the cellular origins, anatomy, transmitter contents, and, where known, the actual functions of the different components of the developing nervous systems.
Even with the exclusion of molecular biology, however, full coverage of invertebrate neural development in a single chapter is utterly impossible. The reader is therefore also referred to other sources for background literature. As a first step for readers with only a limited background outside the mammals or even the vertebrates, Pechenik (2005) offers a thoroughly readable (in fact, enjoyable) introduction to invertebrate biology, while a more detailed overview is offered by Brusca and Brusca (2003). For a glimpse at the intricacy, diversity, and beauty of the different larval forms, the reader is referred to Young, Sewell, & Rice (2002). For more focused overviews of invertebrate development, larval biology, and evolution of larval forms, the reader should consult Gilbert and Raunio (1997), Hall and Wake (1999), and Nielsen (2001).
A recurring theme of this chapter will be the (often uncertain) evolutionary relationships between different animal groups. The following sections will be organized into a scheme which groups the simplest animals, the diploblasts, and divides the more complex bilaterian invertebrates among three clades: Lophotrochzoa, Ecdysozoa, and Deuterostomia.
11.2 Basal Diplobalastic Metazoa with Nervous Systems
Together with their roughly radial symmetry, the defining characteristic of the diplobastic phyla, Cnidaria and Ctenophora, is their possession of only two germ layers, an outer ectoderm and inner endoderm. The layers are then generally separated by a loose extracellular matrix forming the mesoglea.
11.2.1 Cnidaria (Including Jellyfish, Corals, Anemones)
The Cnidaria are illustrative of many of the complexities encountered in comparative studies of neural development across the animal kingdom. As a sister clade to the Bilateria, the Cnidaria have long been the subject of close scrutiny with regards to the structure and function of the adult nervous system. However, while these animals are relatively simple in terms of overall structure, their life cycles are complex and large differences exist across the phylum. In the general developmental plan of Cnidaria, planula larvae metamorphose into the sessile polyp stage which then divides asexually to produce either additional polyps or numerous swimming medusa stage individuals (see Figure 11.1A). The medusae provide the next generation of planulae through sexual reproduction. There are, however, large variations in this plan. In the Sciphoza (true jellyfish) and Cubozoa (box jellyfish), the medusa stage dominates, while in the Hydrozoa (hydroids), the polyp stage tends to be the most noticeable. In the Anthozoa (anemones and corals) the medusa stage is lost entirely. In addition, the early development of Cnidaria is variable, with cleavage ranging from radial to irregular, unequal, or superficial, eventually giving rise to a blastula which, in turn, undergoes mixed patterns of ingression, invagination, delamination, and epiboly during gastrulation (Martin, 1997). Such variations make lineage studies difficult to generalize. Given such large differences in development it is not surprising that findings from different species seem contradictory, although broad generalities are emerging.

Figure 11.1 Larval Forms of Cnidaria and Flatworms.
(A) The planula larva is typical of the cnidaria, some of which (i.e., the Scyphozoa) also transit through a scyphisoma polyp stage before assuming an adult medusa form. (B) The miracidium and cercaria stages are found in different hosts of parasitic trematode flatworms. (C) The Müller’s larva is one of the forms found in polyclad Turbellaria flatworms.
Modified from Pechenik 2005. Reproduced with permission of McGraw‐Hill Companies, Inc.
Neurogenesis commences late in, or shortly after, gastrulation and produces two broad categories of nerve cells. Sensory cells appear to transdifferentiate from epitheliomuscle cells and remain in situ to span the epithelium from the mesogleal surface to the free surface of the planula, where they bear ciliate or microvillus endings (Martin, 1988; Piraino et al., 2011). Some sensory cells also appear dark and some light under electron microscopy (EM), and a portion contain detectable levels of RFamide neuropeptides (Martin, 1988). Ganglion cells, on the other hand, differentiate from a type of pluripotent stem cell known as the interstitial cell (i‐cell), although this progenitor cell type may be missing in some cnidarians (Marlow, Srivastava, Matus, Rokhsar, & Martindale, 2009). Extensive migration may occur before the ganglion cells differentiate to form both the somata and the processes located at the base of the epithelium and constituting an extensive network adjacent to the mesoglea. The ganglion cells thus appear to function as interneurons with connections to sensory cells and other ganglion cells and with possible release of transmitters (e.g., catecholamines, serotonin, taurine, and GABA in addition to RFamide and GLWamide peptides) into the mesoglea. Some processes of the ganglion cells have also been reported to project to the outer surface of the planula, suggesting a possible sensory function for some of these cells as well (Martin, 1988, 1992, 1997).
Although the nervous system of the planula is often described as a diffuse network, an anterior/posterior polarity is commonly reported. For example, Martin (1992) reported concentrations of RFamide containing cells at the anterior (aboral) end, a finding that has been confirmed in other species (Marlow et al., 2009; Piraino et al., 2011). Tightly packed, elongated sensory cells appear to form a discrete apical organ, which may be replete with an apical tuft of cilia in some species (Marlow et al., 2009; Yuan, Nakanishi, Jacobs, & Hartenstein, 2008). Piraino et al. (2011) suggest that polarization may be most extreme in species with planulae that crawl in wormlike fashion rather than swim like most species.
This concentration of peptides at the anterior end of the swimming larvae disappears following settlement and metamorphosis, which involves a fundamental change from swimming to sessile forms with the attachment of the previous anterior end of the larva to the substrate. The previously posterior end of the animal then forms tentacles around the mouth/anus, which in the polyp, as in the planula, is the sole opening into and out of the gastric cavity. The polyp has a nervous system reminiscent of the planula with a diffuse nerve net made of sensory and ganglion cells, although neuronal elements are concentrated into a distinct ring around the mouth (oral ring) and in the tentacles. While neurons can be observed in regions of the endoderm in late stage planulae, they become much more numerous in the polyp.
11.2.2 Ctenophores (Comb Jellies)
The ctenophores have long been recognized as basal metazoans, with body plans and molecular characteristics that provide insight into the first nervous system—nonetheless, in comparison to the cnidaria, they remain poorly studied. It has been estimated that the roughly 200 known species of ctenophores represent only about 25% of the total number in nature, with the majority of undescribed species residing deep in the oceans (Martindale & Henry, 1997) and unsampled by traditional collection through trawling (Pechenik, 2005). Even studying the basic biology of species that dwell near shore is hampered by difficulties in rearing and maintaining specimens in aquaria and by the delicacy of their tissues, which often disintegrate when placed in common fixatives (personal observations). Hence, little is known about the adult nervous system (Jager et al., 2011) and still less about development.
Early cleavage in ctenophores follows neither the spiral nor radial patterns commonly observed in other animals and provides one of the most striking examples of determinate mosaic development, with experimental separation of individual blastomeres at the two cell stage producing two nearly perfect half animals. The eight cell stage consists of four E and four M blastomeres with the first micromeres produced from these cells (the e1 and m1 micromeres) being the source of the nerve net, which begins to form shortly after gastrulation by epiboly (Martindale & Henry, 1997).
The typical larva of the ctenophores, called a “cydippid,” has eight comb rows and two tentacles. Larvae possess a nerve net and apical organ similar to the adult, but details are lacking. Cydippid larvae closely resemble the adult form in species such as Pleurobrachia, and one could argue that there is no true metamorphosis (Martindale & Henry, 1997). In other species, however, tentacles are lost, lobes grown, or the bodies changed from globular to wormlike. With the paucity of information currently available regarding neural development in this important phylum, the field is clearly wide open for future study.
11.3 Lophotrochozoa
The Lophotrochozoa form the largest clade of protostome animals in terms of numbers of phyla. The name signifies an amalgamation of animal groups characterized by either lophophorate or trochophore larval types (see below); modern molecular evidence also includes Platyzoa. While evidence indicates lophotrochozoans are monophyletic (Philippe, Lartillot, & Brinkmann, 2005), they can differ even with regard to such basic characteristics as early cleavage patterns (i.e., spiral and nonspiral), and relationships between subgroups are matters of constant discussion (Giribet, 2008; Philippe et al., 2005).
11.3.1 Platyzoa
The Platyzoa include the platyhelminthes, gastrotrichs, rotifers and assorted minor phyla, but only the platyhelminths are discussed below. While inroads are being made into understanding the adult nervous systems of other phyla (Hochberg, 2007; Hochberg & Lilley, 2010), little is known about their neural development.
11.3.1.1 Platyhelminthes (flatworms).
Because of their simple body plan, together with other special features such as the lack of a coelum, the platyhelminthes had long been considered as a basal sister group to the rest of the Bilateria. However, molecular studies and re‐evaluations of early cleavage patterns now favor a position for this group within the Lophotrochozoa (Ellis & Fausto‐Sterling, 1997; Giribet, 2008; Rawlinson, 2010). Hypotheses regarding the form of the ancestral flatworm larvae are complicated by phylogenetic uncertainty (Egger et al., 2009; Rawlinson, 2010). Additionally, life histories of the different groups of flatworm are extremely complicated, with three of the four classes showing dramatic adaptations to parasitic lifestyles. For example, many trematodes (flukes) and cestodes (tapeworms) possess sequential larval forms corresponding to different animal hosts (Figure 11.1B). Even within the turbellarians, which have free‐living species and appear to best represent the ancestral forms, development can be highly variable; some species hatch as planktonic larvae (for example, as eight‐lobed Müller’s larvae (Figure 11.1C), four‐lobed Götte’s larvae, or larvae with other numbers of lobes), others retain features of larval forms embryonically, but hatch as juvenile benthic worms, while yet others manifest few if any larval characteristics as embryos before hatching as juveniles.
A final complication hindering comparisons of ontogeny of different flatworms lies in the special characteristics of the eggs and corresponding cleavage patterns (Ellis & Fausto‐Sterling, 1997). One group of flatworms (archoophoran) produces oocytes which show early spiralian cleavage (Boyer, Henry, & Martindale, 1998; Younossi‐Hartenstein, Jones, & Hartenstein, 2001) but are surrounded by numerous yolk‐rich nurse cells. The dividing embryonic blastomeres eventually form a consolidated mass of cells which moves toward the surface of the embryo. At this point, cells in deep layers become postmitotic neural and muscle precursors and begin to differentiate. Cells at the surface form an epithelium which then differentiates into the epidermis. Thus, in the neoophorans, the formation of separate epithelial germ layers through gastrulation, which characterize the ontogeny of most other animals groups (including other flatworms), is absent. Despite these differences between archoophoran and neoophoran flatworms, Younossi‐Hartenstein et al. (2001) point out that neurogenesis in both of these groups shares the feature that progenitors of central neurons are not derived from a pre‐existing neurectoderm (see below), but instead are internalized before commitment to a neural fate. Both developmental programs produce similar embryonic nervous systems.
The central nervous system of the embryo and larva consists of an anterior brain that can include between 30 and 50 neurons, depending on the species and presumably related to lifestyle demands upon the young organism (Younossi‐Hartenstein & Hartenstein, 2000). Eyes are often situated directly on the brain. Axons from neurons in the brain project posteriorly in 2–3 pairs of connectives. Contralaterally projecting axons form the regularly spaced commissures, which produces the central nervous system characteristic of flatworms. Some fibers within the connectives of the central nervous system contain serotonin or FMRFamide immunoreactivity in a free‐living turbellarian larva, but other fibers (labeled with antibodies against acetylated tubulin) apparently contain neither of these transmitters. Indeed, several histological and immunocytochemical studies have revealed extensive larval nervous systems in parasitic flatworms by targeting neurotransmitters such as acetylcholine, monoamines, nitric oxide and a variety of peptides (Gustafsson & Terenina, 2003; Kemmerling, Cabrera, Campos, Inestrosa, & Galanti, 2006).
In addition to the anterior brain and posterior connectives which characterize all flatworm larvae and juveniles, the free‐living forms also possess a peripheral nervous system. Lacalli (1982, 1983) used electron microscopy to first describe the detailed ultrastructure of a Müller’s larva and identify the regularly spaced sensory neurons with axons closely associated with the various ciliary bands which generate locomotor drive and feeding currents for the larvae. Lacalli emphasized the independent natures of the intraepithelial peripheral nervous system and the subepithelial central nervous system, a finding that has largely been confirmed by anatomical studies on other flatworm larvae (Rawlinson, 2010). Lacalli’s suggestion that the peripheral sensory neurons differentiate in situ from the surrounding epithelium has also been confirmed (Younossi‐Hartenstein et al., 2000; Younossi‐Hartenstein & Hartenstein, 2000), as has the suggestion that the apical organ in free‐living larvae appears to be composed of central ciliated cells surrounded by flask‐shaped glandular cells, and is thus nonneural, although it may still mediate a sensory function through innervation from the brain (Rawlinson, 2010; Younossi‐Hartenstein, Ehlers, & Hartenstein, 2000; Younossi‐Hartenstein & Hartenstein, 2000).
Metamorphosis in flatworms is gradual with the resorption of lobes and the apparent loss of the intraepithelial sensory neurons and apical organ (Rawlinson, 2010). The number of posterior connectives also generally increases to yield the nervous system typical of the adult flatworm (see Chapter 8).
11.3.2 Lophophorata and Endoprocta
Problematic phylogenetic relationships of many invertebrate groups impede our understanding of the evolution of the nervous system throughout the animal kingdom. The lophophorates have been particularly difficult. These animals have been grouped together based primarily on morphological features of the adults and larvae. However, this group exhibits a bewildering array of embryological and morphological characteristics that have defied even broad categorization into protostomes or deuterostomes. For example, zygote cleavage has long been described as basically radial, and their regulated development is typical of deuterostomes; likewise, Nielsen (1987) has argued that the position and beat direction of cilia generating feeding currents are characteristic of deuterostomes. In sharp contrast, the fact that the mouth of phoronids originates from the blastopore—the defining characteristic of protostomes—argues for inclusion in the latter category. Molecular evidence now places the Lophophorata not only within the protostomes, but close to other Lophotrochozoa (Helmkampf, Bruchhaus, & Hausdorf, 2008), although these findings leave unresolved the mismatch between genetic and morphological/developmental characteristics.
11.3.2.1 Phoronids (horseshoe worms).
Cleavage of the phoronids is biradial and by the 16‐ or 32‐cell stage a small blastocoel has already formed. Gastulation occurs by invagination of the vegetal pole thus resulting in the formation of the germ layers which give rise to the actinotroch larva (Figure 11.2A) typical of the phylum (Zimmer, 1997). Many of the basic features of the larval nervous system were described using light microscopy from the end of 19th century through the middle of the 20th century (reviewed by (Hay‐Schmidt, 1989). Details of the ultrastructure of the larval nervous system were provided at the EM level by Hay‐Schmidt, (1989) and Lacalli (1990) and subsequent immunocytochemical studies provided visualization of discrete populations of neurons containing catecholamines, serotonin or FMRFamide‐related peptides (Hay‐Schmidt, 1990a, 1990b, 1990c; Santagata & Zimmer, 2002). Those descriptions of the larval nervous system emphasized its intraepithelial nature, thus suggesting that early neurogenesis involves only the differentiation of neurons from surrounding epithelial cells without the need for internalization of neural progenitor cells, as often seen later in other animals.

Figure 11.2 Larval Forms of Lophophores.
(A) Actinotroch larvae are typical of the phonorids. (B) Brachiopod also possess a unique larval form. (C) Both cyphonaute and coronate larvae are found in the Bryozoa.
Modified from Pechenik 2005. Reproduced with permission of McGraw‐Hill Companies, Inc.
Some differences occur between species in details of the larval nervous system (Hay‐Schmidt, 1989; Lacalli, 1990; Santagata & Zimmer, 2000), but, generally, cell bodies of the nervous system reside within or surrounding the apical ganglion. Up to four types of ganglion cells, including putative sensory cells and neurons, can be identified based on ultrastructural criteria. Serotonergic cells appear early in development, forming a U‐ or V‐shaped configuration around the central core of neuropile. Later‐appearing cells can occupy more central positions. Several catecholamine‐containing cells also appear early and encircle the neurons containing serotonin. Fibers from all these cells contribute axons to the main nerves, which emanate from the apical organ to innervate the rest of the surrounding epistome and from there the rest of the larva and especially the tentacles with their ciliary bands. FMRFamide‐related peptides are also abundant in the nerves, although somata are only sparsely labeled immunocytochemically. Rows of specialized epithelial cells that were innervated and presumed to be sensory were also found along the tentacles (Hay‐Schmidt, 1989). As the larva reaches competence for metamorphosis, a secondary neuropile forms under the hood organ which also contains putative sensory cells.
Metamorphosis is striking and profound in phoronids, whereby the entire larva turns inside out, apparently through muscular contractions orchestrated by the nervous system (Santagata, 2002). The cells of the apical ganglion undergo cell death, muscles disappear and the gut and tentacles are remodeled in ways that vary between species to produce the adult form.
11.3.2.2 Brachiopods (lamp shells).
The brachiopods are generally thought to be closely related to the phoronids and accordingly their early development is very similar, although since the blastopore closes completely during development, its relationship to the mouth is unclear. The articulate brachiopods produce simple lobed larvae, which do not feed and exist for only a few hours before settlement, yet nonetheless may possess complex musculature and reflexes. These larvae have eye spots and respond specifically to environmental cues for settlement (Zimmer, 1997). As the larvae become competent to metamorphose, they develop prominent flask‐shaped and presumably sensory serotinergic cells in the apical organ; these disappear during metamorphosis (Altenburger & Wanninger, 2010). The inarticulate brachiopods, on the other hand, have larvae (Figure 11.2B) which can swim and feed for several weeks before settlement using cilia located along well‐developed tentacles. The larval nervous systems of Lingula and Glottidia have been described by Hay‐Schmidt, (1992) using EM and immunocytochemistry, and consist of a “ventral” division comprising both the apical organ located within a medial tentacle (Luter, 1996) and the innervation to the lophophore and a “dorsal” division which comprises both the ventral ganglion and the innervation of the body musculature associated with the shells unique to this taxon. The former division compares to the larval nervous system seen in phoronids, whereas the latter is suggested to have evolved only within the brachiopods (Hay‐Schmidt, 1992).
11.3.2.3 Bryozoa (ectoprocta, or moss animals).
The bryozoans are a strange group of animals which, nonetheless, share enough morphological, developmental, and molecular similarities to other Lophophorata to classify them as distant relatives to phoronids and brachiopods (Hausdorf, Helmkampf, Nesnidal, & Bruchhaus, 2010).
Bryozoans have biradial cleavage, and by around the 64‐cells stage they gastrulate through the internalization of the vegetal tier of cells. The tier of eight cells at the animal pole eventually produces the neurons of the apical disc. Some bryozoans, however, exhibit a very unusual form of reproduction (polyembryony), which is poorly studied in its early stages but which involves a single zygote producing several (hundreds of) larvae. More usually, byozoans produce either nearly spherical coronate larvae, which are brooded and hence are short‐lived and nonfeeding, or triangular, shelled cyphonaute larvae (Figure 11.2C) which can swim and feed in the water column for several weeks before settlement (Zimmer, 1997).
The larval nervous system has now been studied in several species, and demonstrates numerous similarities over a wide variety of body forms (Santagata, 2008). One consistent component is the numerous neurons in the neural plate in the center of the apical disc and several more cells in surrounding regions. Unlike many other invertebrate larvae, there is no neuropil underlying the apical cells (but see Wanninger, Koop, & Degnan, 2005); instead, the axons from these cells project to a nerve nodule underlying the pyriform organ near the extreme anterior end of the larva. Nerves projecting from the nexus also connect to other regions of the body and can thus provide innervation to the ocelli, balancer cells, and various other putative sensory organs. Linking form to function, Santagata (2008) noted that innervation of regions involved in feeding were more developed in planktotrophic larvae while the fields of sensory cells in the apical organ were more developed in lecitrophic (yolk‐feeding) larvae. Pires and Woollacott (1997) report the coronate larvae of Bugala are positively phototactic when they are first released but then become negatively phototactic as they prepare to settle: Dopamine, which is detectable in the larvae, prolongs the period of positive phototaxis; serotonin, present in cells in the apical organ and along the edge of the ciliated corona (Gruhl, 2009), causes a rapid shift to negative phototaxis.
The processes mediating metamorphosis vary greatly, as might be expected by the range of larval and adult forms in this phylum, but can involve eversion, inversion, or degeneration of major body regions, including the larval nervous system. While dramatic, these processes must be viewed in a broader context of the rest of the life cycle. The larva metamorphoses into an ancestrula, which founds (by budding) a colony of hundreds or thousands of individuals. The ways that identical adult nervous systems can be derived from either sexual or asexual reproduction are unknown, but are encountered in colonial animals (e.g., corals and colonial ascidians) from other phyla.
One additional and remarkable feature of the bryozoan life history warrants mention. The entire body, except for the thin outer wall of individual bryozoans (or “cystid”), periodically degenerates and is expelled as a “brown body.” A new inner body (the polypide), including the tentacles, gut, musculature, and nervous system, is subsequently regenerated. This process, called polypide replacement, occurs either in response to unfavorable environmental conditions or due to accumulating metabolic wastes in animals that lack nephridia. As mentioned by Zimmer (1997), the ability of bryozoans to regenerate all body tissues from just a few cell types challenges long‐held concepts of triploblastic germ layer specification through gastrulation.
11.3.2.4 Entoprocta (Kamptozoa).
Like the lophophorates, entoprocts are characterized by a ring of ciliated tentacles which surround the mouth and are used for both feeding and respiration. Entoprocts are distinct in that their anus lies within this ring, and that the direction of water currents generated by ciliary beating is opposite to that in lophophorates. Molecular evidence has been limited, since these animals are not often represented in large‐scale phylogenetic analyses (but see Hausdorf et al., 2010). Hence, these animals are sometimes hypothesized to have closest affinities with bryozoans or molluscs, with much evidence based on morphology and development.
Distinctly unlike the lophophorates, the cleavage of entoprocts is spiral and determinate. Moreover, the swimming larvae of some entoprocts have a trochophore‐like form. Hay‐Schmidt, (2000) provided an initial description of the larval nervous system of an entoproct, but more complete descriptions were later provided by Wanninger, Fuchs, & Haszprunar (2007), who examined developing serotonin‐like immunoreactivity and Haszprunar and Wanninger (2008), who employed EM. Both studies reported larval nervous systems with similarities to basal molluscs. Specifically, the creeping‐type larva of Loxosomella was reported to have a complex apical organ containing about eight central and an equal number of peripheral serotonergic neurons. Two ventral and two lateral nerve cords projecting posteriorly from the cerebral ganglion were also reported, as seen in some molluscs and polychaetes. Another similarity was a prototrochal nerve ring, although it was pointed out that the arrangement of ciliary bands on molluscs and entoprocts did not support direct homologies. These types of similarities were sufficient for both studies to suggest strong affinities between the molluscs and the entoprocts.
Many entoprocts, like the bryozoans, form colonies through asexual budding, and Wanninger, Fuchs, Bright, & Funch (2006) reported that neurons expressed serotonin and FMRFamide like immunoreactivity very early during bud formation. The fact that no connections were detected between the nervous systems of the adult and the bud suggests the new nervous system differentiates independently, but further details are lacking.
11.3.3 Trochozoa
This taxa’s characteristic trochophore larva (see Figure 11.3) possesses several ciliary bands and an apical organ associated with its own ciliary tuft. Occasional eye spots and tentacles can be found in the episphere, which is demarked caudally by the prototroch, a ciliary band which generates locomotion. The caudal end of the larva (the hyposphere) contains another ciliary band, the metatroch, a ventral mouth between the prototroch and the metatroch, and a caudal‐most anus, often associated with a third ciliary band, the telotroch. Some trochozoans (e.g., the polychaete annelids) also possess an additional longitudinal band of cilia, the neurotroch, along what will become the ventral surface. Many trochozoans metamorphose directly from the trochophore into a juvenile form, while others display intermediate forms, such as the veligers (Figure 11.3) of gastropods and bivalves or the elongated and already‐segmented metatrochophore of the polychaetes. The similarity of larval structures and development across this group, and particularly between the annelids and molluscs, have generated interest in possible common functions of organs (e.g. Voronezhskaya & Khabarova, 2003; Nielsen, 2005) and developmental mechanisms (Voronezhskaya & Ivashkin, 2010).

Figure 11.3 The Trochophore Larva Characteristic of the Trochozoa.
Some molluscs, such as the polyplacophora (chitons) and Scaphopoda (tusk shells) metamorphose from the trochophore into a juvenile stage while the gastropods and bivalves generally have an intermediary veliger stage. Polychaete annelids also have an elongated late larval stage referred to as a metatrochophore.
Modified from Pechenik 2005. Reproduced with permission of McGraw‐Hill Companies, Inc.
11.3.3.1 Annelids (segmented worms).
Neural development has been studied extensively in two groups of annelids, the leeches and the polychaetes, which differ profoundly from each other in several aspects of ontogeny.
The leeches are a highly derived group of annelids which develop within their egg capsules to hatch as miniature, sexually immature versions of the adults. They have thus lost the larval trochophore stage. The large and identifiable cells of the adult CNS, however, have been widely studied and offer well‐defined endpoints for developmental investigations. Thus leeches illustrate the development of single cells from their first specification as neurons to the point that they attain their adult phenotypes with stereotyped positions, sizes, transmitter and receptor complements, and axonal morphologies. Furthermore, the development of behavior can be studied in terms of the assemblages of mediating circuitry. These advantages served as incentives propelling leeches into being among the earliest modern subjects of neural development (Stent, Kristan, Torrence, French, & Weisblat, 1992).
As in other annelids, cleavage in leeches is highly stereotyped (Stent et al., 1992). After three cleavages the zygote has produced four macromeres (A,’ B,’ C,’ D’) and the first quartet of micromeres (a,’ b’, c,’ d’). The anterior supraesophageal ganglion is derived from this and other early quartets of micromeres. Subsequent divisions of the D‘ macromere produce bilateral pairs of large stem cells which are referred to as M, N, O, P, (or two roughly equivalent O/P) and Q teloblasts. Asymmetric divisions of these teloblasts produce longitudinal bandlets of n, o, p, and q ectodermal blast cells which constitute the germinal plate and eventually form the ventral nerve cord. Underlying mesodermal m blast cells primarily become muscles. Intracellular dye injections have demonstrated that all four ectodermal teloblasts, and even the mesodermal teloblast, contribute to the segmental ganglia of the ventral nerve cord. The majority of neurons in the segmental ganglia derive from the n bandlet, while the o, p, and q bandlets produced greater proportions of peripheral neurons. The relationship between developmental ancestry and different neuronal attributes is complicated, however, and Stent et al. (1992) concluded that, despite substantial migration of neuroblasts and immature neurons, ancestry correlated best with final positions of neurons in the nervous system and not with other neuronal attributes, which appear to be determined by cell‐to‐cell interactions.
While the development of the adult annelid nervous system (e.g., anterior ganglia and ventral nerve cord) has been best studied in the leeches, recent research adds a comparative perspective by also describing neural development in the polychaetes. Some information is complementary. For example, Denes et al. (2007) described cellular events and gene expression patterns during the formation of the ventral nerve cord from a neurectoderm in Playnereis and described many similarities with development of CNS in vertebrates. Meyer and Seavers (2009) provided further details of neurogenesis by focusing on cellular events during neural differentiation and internalization of neuronal precursors that form the anterior brain (cerebral ganglia) of another polychaete, Capitella.
However, in contrast to the leeches, the polychaetes have a distinct trochophore stage and a larval nervous system which permits a free‐living mode of existence at this early period of development. Lacalli (1984) made pioneering EM studies and described the larval nervous system as appearing much earlier than the first rudiments of the adult brain and nerve cord and as being composed of two parts. The pretrochal component comprises the apical organ and a few other neurons in the episphere and innervates the prototroch, the anteriormost of the locomotor ciliary bands. The apical organ itself was described as comprising about 16 neuronal and nonneuronal cells, most of which possessed ciliary surface specializations. The second component comprises neurons innervating the mouth regions and metatroch. Lacalli (1984) emphasized that this larval nervous system was largely independent of the later‐developing adult nervous system, although he also noted that the cerebral commissure of the adult nervous system became intimately associated with the apical organ and that the apical organ may play a role in organizing the development of the commissural fibers.
More recent immunocytochemical studies have revealed additional cells, added details to the earlier descriptions, and showed that many of the larval neurons exhibit serotonin or FMRFamide‐like immunoreactivity. These studies have permitted a fuller view of the developmental sequence in the assemblage of the larval nervous system, but have also revealed needs for additional studies of underlying developmental processes. For instance Voronezhskaya, Tsitrin, & Nezlin (2003) and McDougall, Chen, Shimeld, & Ferrier (2006) demonstrated that the very first neurons to develop in the larvae of different species of polychaetes were not located in the apical region, as would have been consistent with earlier work on other trochophore larvae, but at the posterior end of the larva. The functions of these serotonin‐containing posterior cells, and numerous other peripheral neurons, are generally unknown and warrant further investigation. In addition, Hay‐Schmidt, (1995) viewed later neural development as a transformation of the larval nervous system into the adult nervous system, whereas Voronezhskaya et al. (2003) argued that certain larval neurons formed a framework or scaffolding of pioneering fibers upon which the adult nervous system was built, before disappearing with the rest of the larval nervous system at metamorphosis. Another important contribution of later immunocytochemical studies is that they have examined a diversity of species and demonstrate major differences in details of early neuronal development in the polychaetes, supporting the notion that the polychaetes are themselves diverse and perhaps paraphyletic (Brinkmann & Wanninger, 2009).
Although details differ, studies on larval polychaete nervous systems have consistently revealed a surprisingly complex and extensive nervous system in what was once viewed as a simple larval form. These studies have also provided information on neural circuitry underlying larval behaviors such as phototaxis (Jékely et al., 2008) and vertical migrations in the water column (Conzelmann et al., 2011) and might therefore additionally suggest potential functions of the larval nervous systems in other phyla of Trochozoa.
Near the time of metamorphosis, the trochophore or elongated metatrochophore larvae of polychaetes contains a brain (the cerebral or supraesophageal ganglion, which, as in the leech, derives from early micromeres, primarily the first quartet) and often 1–3 ganglia of nerve cord (derived primarily from later divisions of the D blastomere). After metamorphosis, the body elongates by sequential additions of posterior segments during postlarval life. The timing of neurogenesis in the nerve cord is thus very different between the leech and polychaetes. Specifically, the germinal plate is laid down by continuous and fairly rapid divisions of the blast cells derived from the D blastomere in the embryonic leech. In polychaetes, however, the segmental ganglia are laid down one by one over a more extended time, and are derived from teloblasts which remain active in a proliferative zone, pygidium, near the anus.
11.3.3.2 Echiura (spoon worms).
Echiura constitute a small taxon of only about 150 species which burrow into the mud in shallow marine habitats. Their common name derives from the flattened proboscis with which they feed. The echiurans have generally either been classified as a separate phylum closely related to the annelids or as a subtaxon within the annelids. Recent molecular evidence favors the latter relationship (Struck et al., 2007).
Cleavage is spiral in echiurans, and an annelid cross (rotated 45o from the typical cross of molluscan and sipunculan development) forms around the apical rosette by the 48‐cell stage. Gastrulation occurs either by proliferation of animal micromeres and epiboly or by invagination of the vegetal plate. As with annelids, the trochophore larvae of echiurans possess bands of cilia arranged into a prototroch and telotroch, and also neurotroch and metatroch in some species (Pilger, 1997).
Different species of echiurans possess early larval nervous systems associated with the pharynx and the various ciliary bands (Hessling, 2002; Hessling & Westheide, 2002). No evidence for an apical organ was reported, and the first neurons appeared in circumesophageal ganglia. The focus of these studies was the detailed description of ventral nerve cord development, since the lack of demonstrated ventral nerve cord segmentation had been a major argument against including echiruans among annelids. Hessling (2002) and Hessling and Westheide (2002) exploited immunocytochemistry and confocal microscopy to show ventral nerve cord segmentation in these animals during larval stages with serial reiteration of serotonergic and FMRFamidergic elements and tubulin‐rich axonal tracks. Furthermore, as in annelids, the nerve cord is initially a bilaterally paired structure which develops in an anterior‐to‐posterior sequence. These features are lost or obscured in the adult (Pilger, 1997; Hessling, 2002).
A final noteworthy feature of echiuan development involves the profound sexual dimorphism that occurs in some species (Pilger, 1997): The adult males in these animals live inside the reproductive tracts of the females, upon which they depend for nutrition and protection. Accordingly, post‐metamorphic development of females is associated with an enlarged proboscis and trunk and corresponding changes in the nervous system. In contrast, post‐metamorphic development of males appears to involve only elongation of the trunk, without corresponding changes to the nervous system (Hessling & Westheide, 2002).
11.3.3.3 Sipuncula (peanut worms).
Sipunculans are wormlike animals that have variously been aligned with either annelids or molluscs. Cleavage is spiral, and when the embryos reach the 48‐cell stage, their apical plate forms a molluscan cross pattern, even though recent molecular evidence favors a closer relationship with annelids (Wanninger, Koop, Bromham, Noonan, & Degnan, 2005). The fates of the early blast cells appear consistent with those of other spirilians (Pilger, 1997). Gastrulation can occur through the processes of epiboly, invagination or both. Although some direct developing species lack any larval form, most sipunculans develop through either a single trochophore phase or a trochophore followed by an prolonged pelagophera stage (Rice, 1975).
Wanninger, Koop, Bromham, et al. (2005) originally reported that one sipunculan, Phascolion, possessed no serotonergic neurons in the larval nervous system and no obvious signs of segmentation in either the early nervous system or musculature. That species, however, has an unusually reduced larval stage; examining Phascolosoma, Kristof, Wollesen, & Wanninger (2008) found an extensive larval nervous system innervating the prototroch, apical organ, and mouth region, in addition to the anlage of the adult brain. In contrast to the earlier study, they also reported that the early larval nervous system exhibited a metameric organization with repeated FMRFamide and serotonin immunoreactivity in both neuronal cell bodies and commissures, thus resembling neural development in annelids; in late‐stage larvae, many neurons disappear or migrate, and these signs of segmentation are lost. During metamorphosis, which can last several weeks, the metatrochal cilia are lost. Lacking propulsive force for locomotion, the larva sinks to the bottom and assumes the benthic life of the juvenile and adult. The basic features of the peptidergic component of the nerve cord survive metamorphosis and serve as a foundation for subsequent postlarval development (Wanninger, Koop, Bomham et al., 2005). Notably, even though the early larval nervous system of certain sipunculans exhibit metameric organization, the adult peanut worm shows no sign of segmentation.
11.3.3.4 Molluscs.
Molluscs are the second largest phylum in the animal kingdom in terms of number of species, and their possession of a classical trochophore larva (see Figure 11.3) places them firmly within the Lophotrochozoa sensu stricto. Their spiral cleavage pattern of development has been studied for over a century (Conklin, 1897) and, like other spiralians, they derive their anterior nervous system (larval apical and adult cerebral ganglia) from the first quartets of micromeres, and the more posterior portions of the nervous system develop with at least some contributions from the 2D blastomere. Recently, however, Hejnol, Martindale, and Henry (2007) have added important details and corrections to the earlier fate maps, showing which specific cells of the apical organ arise from individual micromeres and demonstrating that the second quartet of micromeres also contributes components to the posterior portions of the adult nervous system.
An extensive literature describes the larval nervous system across a wide variety of molluscs. The cells of the apical organ are among the first neural elements to be detected during development, appearing shortly after gastrulation. Detailed morphology of various cells of the apical organ were first described using EM (Bonar, 1978; Chia & Koss, 1984; Marois & Carew, 1997a; Page & Parries, 2000; Page, 2002) and more recently using immunocytochemistry. The number and arrangement of vase‐shaped, presumably sensory, apical cells are similar across the molluscs. For example, three serotonergic cells often first appear as the larvae enter the trochophore stage (Croll, Jackson, & Voronezhskaya, 1997; Kempf, Page, & Pires, 1997; Marois & Carew, 1997a, 1997b; Page & Parries, 2000; Voronezhskaya, Nezlin, Odintsova, Plummer, & Croll, 2008) while many gastropods, bivalves, scaphopods and polyplacophorans have additional vase‐shaped cells which contain catecholamines, neuropeptides and nitric oxide synthase (Croll, 2006; Croll & Voronezhskaya, 1996; Dickinson & Croll, 2003; Hens, Fowler, & Leise, 2006; Kempf, Chun, & Hadfield, 1992; Voronezhskaya & Elekes, 2003; Voronezhskaya, Hiripi, Elekes, & Croll, 1999). The apical organs of molluscs also commonly contain cells that have been suggested to be interneurons (Croll, 2006; Friedrich, Wanninger, Bruckner, & Haszprunar, 2002; Kempf et al., 1997; Voronezhskaya, Tyurin, & Nezlin, 2002).
Neurites projecting from the apical cells end in underlying neuropil, or project to peripheral regions of the veliger. Serotonergic varicosities lie in close proximity to ciliated cells of the velum (Croll, 2006; Croll et al., 1997; Dickinson & Croll, 2003; Marois & Carew, 1997c; Voronezhskaya, Nezlin, Odintsova, & Plummer, 2008) and Braubach, Dickinson, Evans, and Croll (2006) showed that serotonergic input enhances ciliary beating on the velum, thus increasing both locomotion and feeding of gastropod larvae. The apical organ has also been suggested to be involved in the transduction of an environmental signal which initiates metamorphosis (Couper & Leise, 1996; Froggett & Leise, 1999; Hadfield, Meleshkevitch, & Boudko, 2000; Hens et al., 2006; Leise & Hadfield, 2000), although other evidence argues for alternative or additional roles for this structure (Kuang, Doran, Wilson, Goss, & Goldberg, 2002; Voronezhskaya, Khabarova, & Nezlin, 2004; Wanninger & Haszprunar, 2003).
Posterior neurons immunoreactive for FMRFamide can also be observed in the early trochophore larvae and have axons that project anteriorly to the apical organ (Croll & Voronezhskaya, 1996; Dickinson, Nason, & Croll, 1999; Dickinson et al., 2000; Dickinson & Croll, 2003). Although such cells appear to be a general feature of gastropod larvae (Cummins, Tollenaere, Degnan, & Croll, 2011), their presence in other molluscs is less consistent (Friedrich et al., 2002; Voronezhskaya et al., 2002; Wanninger & Haszprunar, 2003), and lateral, pretrochal cells might play analogous roles in bivalve larvae (Voronezhskaya et al., 2008). One such role could be to innervate larval muscles (Dyachuk & Odintsova, 2009; Evans, Dickinson, & Croll, 2009; Page, 1997; Wanninger et al., 1999). Indeed, Braubach et al. (2006) reported that both applications of FMRFamide increased the frequency of velar contractions. Another potential role for the early peptidergic cells involves the pioneering of pathways to scaffold adult nervous system development (Croll & Voronezhskaya, 1996; Voronezhskaya & Ivashkin, 2010).
A final category of neurons known to develop early in molluscan ontogeny are the catecholaminergic cells that appear around the mouth of both gastropod and bivalve larvae (Croll, Boudko, & Hadfield, 2001; Croll et al., 1997; Dickinson & Croll, 2003; Dickinson, Croll, & Voronezhskaya, 2000; Voronezhskaya et al., 1999), perhaps suggesting control of different aspects of feeding. Many catecholamine‐containing cells and axons have also been found associated with ciliary bands along the velum and may have an inhibitory effect on locomotion and feeding (Beiras & Widdows, 1995; Braubach et al., 2006).
During the mid‐larval stage, many populations of neurons continue to grow in number and complexity, but this period is also marked by the first appearance of structures that persist into adulthood (e.g., eyes, tentacles, foot, and various retractor muscles) and of the ganglia that form the adult central nervous system. Mid‐larval life thus marks a time when the early larval and the developing adult nervous systems must operate in concert to produce the coordinated final output upon which the free‐living larvae depend for survival. Several previous investigations have focused on the development of the ganglia to understand the origins of identifiable neurons in the adult. Such studies used EM and radioactive birth‐dating techniques (Jacob, 1984; Kandel, Kriegstein, & Schacher, 1981; Schacher, Kandel, & Woolley, 1979) to confirm reports that ganglia derive from specific proliferative zones in the body wall (Raven, 1966). After the initial ganglionic anlagen separate from the proliferative zones, they continue to generate postmitotic neurons that migrate inward to the developing ganglia (McAllister, Scheller, Kandel, & Axel, 1983).
During metamorphosis, in preparation for the adult’s benthic lifestyle, the bands of cilia used for larval locomotion and feeding and the nerve cells that innervate them are lost. The posterior peptidergic neurons and the apical organ disappear during or soon after metamorphosis in numerous species (Dickinson & Croll, 2003; Lin & Leise, 1996; Marois & Carew, 1997b), as do the posterior, peptidergic cells (Croll & Voronezhskaya, 1996; Dickinson & Croll, 2003), apparently via
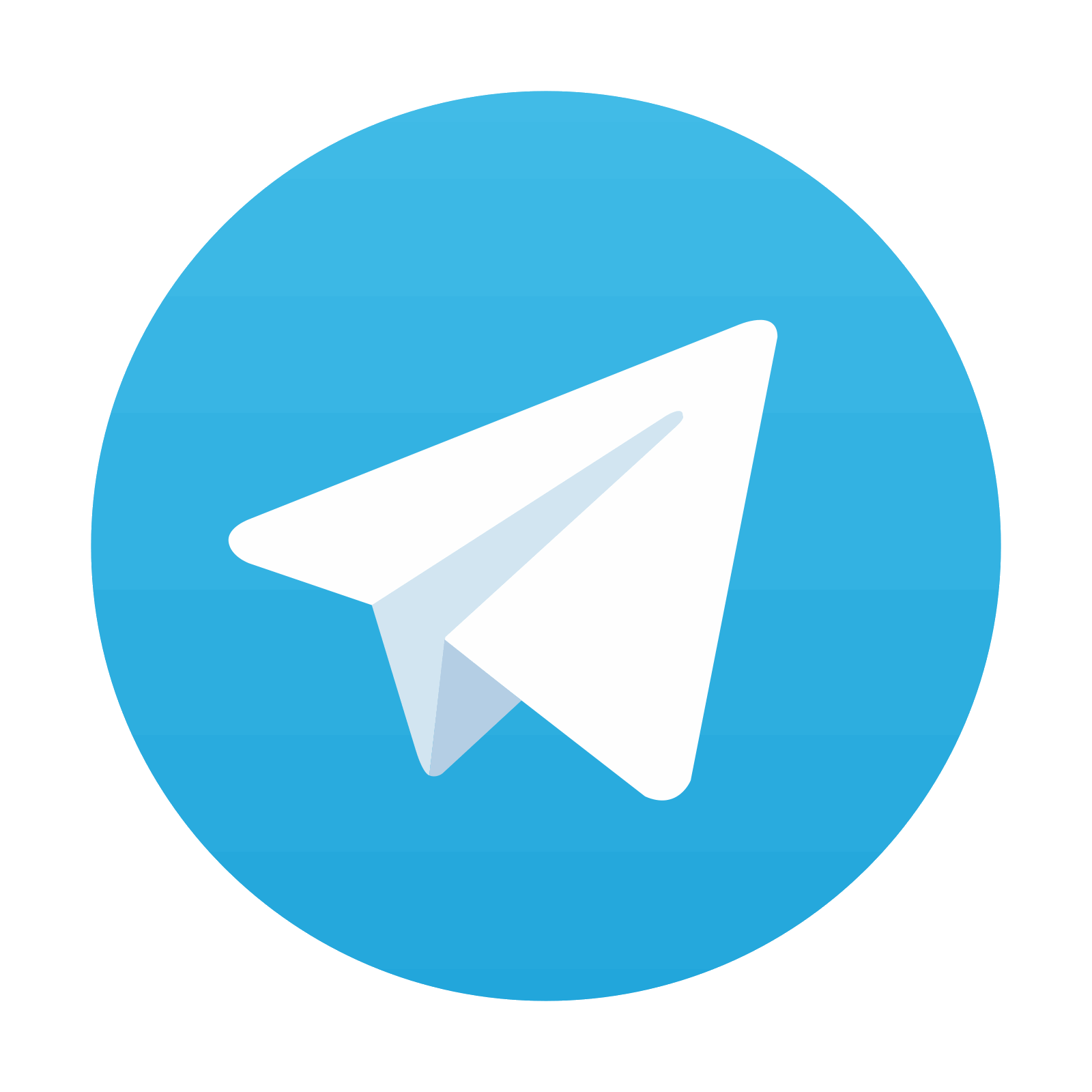
Stay updated, free articles. Join our Telegram channel
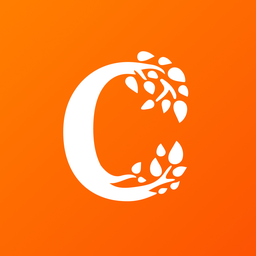
Full access? Get Clinical Tree
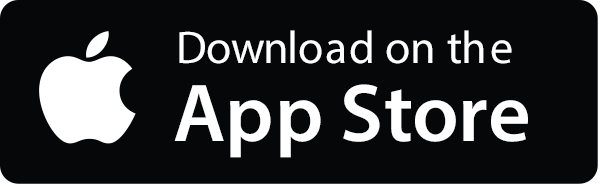
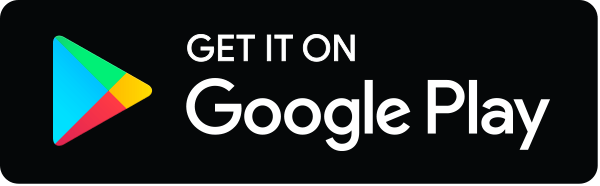