Social Coordination: From Ants to Apes
Anne Böckler, Anna Wilkinson, Ludwig Huber, and Natalie Sebanz
17.1 Introduction
Coordinating actions with others has significant benefits and allows individuals in groups to achieve outcomes they could not achieve on their own. At the same time, living in groups comes with many challenges that are not encountered by solitary individuals. In the last decade, researchers have become more and more interested in the processes underlying different kinds of coordinated group behavior (e.g., Couzin & Krause, 2003; Sebanz, Bekkering, & Knoblich, 2006; Semin & Smith, 2008; Van Overwalle & Baetens, 2009). This ranges from the entrainment and synchronization of actions (Marsh et al., 2009; Schmidt et al., 2011) to the performance of complementary actions in the context of distributed roles (Knoblich, Butterfill, & Sebanz, 2011; Knoblich & Sebanz, 2008). Coordination can rely on informational couplings that occur spontaneously and increase the probability of coordinated behavior, independently of joint plans. However, individuals sometimes also plan their own part of an action in relation to others’ actions, guided by mental representations of desired joint outcomes.
Given the broad scope of coordination phenomena and their underlying mechanisms, understanding coordination clearly asks for an interdisciplinary approach. One ingredient in such an approach is a comparative analysis of coordination in different species. What coordination problems do animals that live in groups confront, what mechanisms underlie the solutions to these coordination problems, and what are the benefits? Coordination problems refer to requirements of social situations in which individuals in a group need to implement or maintain particular relations between their actions. With the present overview we aim to provide a comparative perspective on coordination, exploring whether common solutions to particular coordination problems—such as moving in a group, distributing tasks, coordinating actions in time, and transmitting information—exist across different species. This review thus complements prior work on cooperation (Clutton‐Brock, 2009; Dugatkin, 2002; Gigerenzer, 2008) which addressed principles underlying the exchange of resources and services. The study of coordination tries to understand how individuals in a cooperative or neutral (i.e., non‐competitive) context adjust their actions to each other in time and space.
We do not aim to provide a comprehensive overview of coordination mechanisms in all existing species and neither can we draw any final conclusions on how such mechanisms evolved. Rather, we will illustrate similarities in the ways some common coordination problems are tackled, drawing on studies that include a range of different, mostly group‐living species. As such, this overview provides a basis for speculating on the role of convergent evolution in particular coordination mechanisms and on the cognitive abilities needed to engage in more complex joint actions, such as flexible task distributions or active teaching.
17.2 Moving in Groups
Group motion is a paradigmatic case of coordination. Some group‐living animals can cover great distances by marching, flying, or swimming together for up to several months (Alerstam, Hedenstrom, & Akesson, 2003; Ran et al., 2008). On a smaller time scale, individuals within a group manage to get away from predators by moving rapidly, yet unpredictably. To successfully move as one, two main tasks have to be accomplished by crowds, herds, flocks, and schools: Individuals have to achieve and maintain a stable formation and they have to be able to quickly and flexibly respond to sudden changes in the environment by adjusting their movement direction.
17.2.1 Formation
Traveling with a large number of conspecifics requires individuals to move in a manner appropriate to maintaining group cohesion whilst avoiding collision. Moving groups are often too large for any one individual to survey the complete group and in many cases, there is not one individual guiding or leading the others.
Despite the apparent complexity of this task it appears that simple and general principles control individual behavior. The local interaction hypothesis (Couzin & Krause, 2003; Reynolds, 1987) suggests that, rather than keeping track of the entire group, an individual merely aligns its movements with the movements of surrounding individuals. For example, starlings (Sturnus vulgaris) adjust their flight pattern to that of six to seven neighbors, irrespective of the distance between them and independent of the size of the flock (Cavagna et al., 2007). In locusts (Schistocerca gregaria), alignment of movements increases with increasing density of individuals (Buhl et al., 2006).
Local interaction seems to underlie mammalian herding (Krause & Ruxton, 2002) and much of human crowd behavior as well (Dyer et al., 2008; for a comprehensive review of herding in humans, see Raafat, Chater, & Frith, 2009). Individual wildebeest, for instance, line up direction and speed of movement with that of the herd by simply regulating the distance to neighboring individuals (Chao & Levin, 2007). Typical walking patterns in human pedestrians also emerge from local interactions among individuals of the walking group. When a group of people deliberately walks together (families or friends), group motion can additionally be adjusted to enable communication: Low‐density supports walking side by side, while increasing density fosters V‐like patterns that facilitate conversation (Moussaïd, Perozo, Garnier, Helbing, & Theraulaz, 2010).
By every individual aligning its movement speed and direction with those of surrounding others, groups can form stable self‐organized configurations that do not necessitate signaling (e.g. vocalizations) or a stable group leader. In species characterized by dominance hierarchies, leaders can initiate group movements and modulate their directions or durations while principles of local interaction still hold (Petit, Gautrais, Leca, Theraulaz, & Deneubourg, 2009). For instance, in capuchin monkeys, Cebus capuchinus, collective movements follow principles of self‐organization despite movements being initiated by single individuals (Petit et al., 2009). It seems that different individuals can take the lead in different instances. Group motion, hence, is based on principles of collective movement and can be influenced by individual leadership.
Moving in formations allows individuals in groups to save energy by making use of aerodynamic principles: When flying in formations, pink‐footed geese, (Anser brachyrhynchus: Cutts & Speakman, 1994); pelicans (Pelecanus onocrotalus: Weimerskirch, 2001); and ducks (Anus platyrhynchos: Fish, 1995) appear to save up to 30% of the energy they would need to invest when moving alone. Tuna fish take advantage of induced velocity fields of others’ tail strokes by traveling in diamond‐shaped formations which allows them to reach a higher speed than an individual could achieve alone (Stöcker, 1999). It has been suggested that similar principles are made use of by professional runners or cyclists who reduce wind resistance and preserve energy by forming groups (Kyle, 1979; Olds, 1998).
17.2.2 Responding to Changes in the Environment
In moving groups, adaptation to sudden changes in the environment requires fast decision making. Few individuals may possess relevant information at a given time (e.g., about the location of a predator), therefore rapid information transmission is crucial. Furthermore, individuals may differ with regard to their preferred movement direction, which necessitates consensus decisions to prevent the group from splitting (Couzin, Krause, Franks, & Levin, 2005).
How does information about movement direction spread across a large group? Evidence from fish (three‐spine stickleback, Gasterosteus aculeatus) suggests that responses to sudden changes in neighbors’ direction are highly nonlinear: If just one individual changes direction the group ignores it. However, the probability of other group members adjusting to sudden changes in direction increases logarithmically as more of their neighbors do (Ward, Sumpter, Couzin, Hart, & Krause, 2008). Thus, the more individuals change direction, the faster information spreads across the whole group. This transmission of information is rapid and efficient and maintains group cohesion without requiring individuals to recognize each other or to employ signals such as vocalizations (Couzin et al., 2005).
In an experiment in which groups of stickleback had to reach consensus over which replica fish to follow, the simple behavioral rule of using the majority’s decision proved efficient in reaching near optimal solutions. Accordingly, an increase in group size improved decision quality (Sumpter, Krause, James, Couzin, & Ward, 2008). Experiments on human crowds revealed that a few informed individuals are sufficient to efficiently change the movement behavior of the group (Dyer et al., 2008). Groups of people in these experiments were instructed to walk within a circular arena. People could walk wherever they wanted, but had to stay together as a group. Some individuals received prior information about where to go without leaving the group. Results showed that informed individuals could guide the uninformed group to the designated target without communication or signaling. When conflicting information was provided to different individuals, the group decided in favor of the majority (Dyer et al., 2008). This pattern suggests that group motion in humans is also based on alignment principles and that consensus decision making on movement directions may rely on similar mechanisms of information spreading as found in other group‐living animals.
A major advantage of moving in groups is that it helps individuals to escape from predators. The increased size and density of the group are protective factors. The more individuals a group contains, the greater the chance that a predator will be discovered by one of them (the “many eyes” theory, see Krause & Ruxton, 2002). Furthermore, singling out and capturing individuals becomes more difficult for predators the more homogenous the individuals in a group are (the “confusion effect,” see Krause & Ruxton, 2002). Animals preying upon fish (Carere et al., 2009), starlings (Krause & Ruxton, 2002), or wildebeest (Chao & Lewin, 2007) make fewer kills when the prey are in larger groups than when they are in smaller ones.
Taken together, simple mechanisms such as local interaction and nonlinear responses to motion changes allow large groups to move as one, rapidly transmit information, save energy, enhance speed, and avoid predation. These processes appear to be common to most group‐living animals and play a crucial role in group motion of fish schools, bird flocks, mammal herds, and human crowds.
17.3 Working Together
In group‐living species, efficient foraging, hunting, or territory protection is often best achieved when tasks are divided between individuals (Anderson, Theraulaz, & Deneubourg, 2002; Sendova‐Franks & Franks, 1999). This part of the chapter will consider the mechanisms underlying the distribution of tasks and the integration of individual contributions.
17.3.1 Distributing Tasks
When transporting large objects, building nests, fighting intruders, or hunting, social animals divide labor, either by partitioning tasks sequentially or by forming teams in which different individuals work simultaneously on different tasks (Anderson & Franks, 2001; Jeanne, 1986; Ratnieks & Anderson, 1999). For example, in termites (Hodotermes mossambicus) “workers” cut grass and others subsequently transport it to the nest (Leuthold, Bruinsma, & Huis, 1976).
How are tasks distributed? In large decentralized groups where individual recognition is largely absent, division of labor can be achieved by fixed roles (Anderson & Franks, 2001). For example, when weaver ants (Oecophylla longinoda) build nests, some individuals hold the leaves, others produce glue, and yet others glue the leaves together (Hölldobler & Wilson, 1983). Some tasks even demand physiological specialization such as nest defense in Pheidole pallidula ants, in which intruders are first pinned down by the “minors” and then decapitated by specially‐enlarged mandibles of the “majors” (Detrain & Pasteels, 1992).
Species living in somewhat smaller, stable dominance hierarchies in which individuals recognize each other also divide tasks by allocating roles, but role distribution is somewhat more flexible (Chase, 2002). Cooperative hunting, for instance, can be performed by all group members (e.g. African wild dogs, Lycaon pictus, see McFarland, 1985) or by “hunting parties” of variable sizes (e.g. Harris hawks, Parabuteo unicinaus, see Bednarz, 1988). The size of the hunting group can be adapted to prey difficulty (Scheel & Packer, 1991) and individuals may take different roles and positions in different attacks, such as lions (Panthera leo) circling and killing prey (Stander, 1992).
Group hunting behavior in some species is initiated by dominant individuals. In wolves, Canis lupus, the alpha pair initiates hunting trips and performs most of the hunts (White, 2001). Since the dominant pair in free‐ranging wolves are usually the ones with most hunting experience (e.g., the parents) this seems a way to enhance success (Mech, 1999). In general, hunting success might be a driving factor in role specialization. Experienced group hunters enhance the likelihood of catching prey by taking stable roles. For instance, evidence suggests that lionesses (Pathera leo) circle prey and stalk it towards waiting group members who then catch and kill it. Even though hunting behavior is flexible and depends on the composition of the group, individuals repeatedly occupy similar roles in the hunting formation. In those who circle and stalk, for instance, pairs of experienced hunters tend to take opposite positions in the circle and enhance hunting success by keeping the prey in between them (Stander, 1992).
Roles can also be entirely specialized as a consequence of individuals’ expertise in performing particular parts. Bottlenose dolphins, Tursiops truncates, for instance, display two separate roles when hunting fish in that there are some individuals forming a barrier and one individual driving prey towards the herding individuals (Gazda, Conner, Edgar, & Cox, 2005). It has been shown that the driving role is always occupied by the same individual. Adopting highly specialized complementary roles enables hunts that are extremely difficult to perform. When chimpanzees, Pan troglodytes, chase and circle small monkeys they perform specialized hunting roles that take up to 20 years to learn (Boesch, 2003; Boesch & Boesch, 1989). A similarly slow time course in acquiring particularly sophisticated hunting behaviors is observed in human hunter‐gatherer societies (Gurven, Kaplan, & Gutierrez, 2006).
Recent evidence from joint action studies in humans suggests that roles in a joint task are distributed depending on relative task difficulty (Vesper, van der Wel, Knoblich, & Sebanz, 2012), so that the individual taking care of the more difficult part of the joint action makes less effort to coordinate than the individual performing the easier task. It has also been shown that certain joint action tasks favor leader–follower distributions, where complementary roles emerge spontaneously in the service of coordination (Richardson, Harrison, May, Kallen, & Schmidt, 2011).
In humans, where many joint actions involve role specialization, evidence suggests that, when tasks are distributed across two coactors, people keep track of the other’s part of the task even when this is not relevant for their own performance (Atmaca, Sebanz, & Knoblich, 2011; Böckler, Knoblich, & Sebanz, 2012; Sebanz, Knoblich, & Prinz, 2003). In these experiments, pairs of participants sit next to each other, each responding to a particular stimulus feature on a computer screen by carrying out a specific action (e.g., one of them pressing an assigned response button when seeing the letter S while the other presses another button when perceiving the letter H). People often show performance patterns similar to when they carry out both parts of the task alone. For instance, when the relevant stimulus letter is surrounded by distracter letters, people perform worse when the distracting letters are linked to the coactor’s response (Atmaca et al., 2011). The same pattern is seen when people carry out the same task alone, but respond to both letters by pressing two different response buttons (Eriksen & Eriksen, 1974). Results like these suggest that people, when performing specialized roles, automatically take into account aspects of the task that are relevant for the other. There is some evidence to suggest that the ability to form representations of a coactor’s task is linked to the ability to attribute mental states to others (Humphreys & Bedford, 2011; Ruys & Aarts, 2010) but future work is needed to clarify what kind and level of mind‐reading is required.
17.3.2 Coordinating Individual Actions
While distributing different parts or roles in joint actions facilitates action planning, many coordinated interactions require fine‐grained temporal and spatial adjustments of individuals’ actions toward each other. When groups are large or decentralized, an important means of coordination is signaling, for instance by leaving chemical traces in the environment. Using pheromones, ants coordinate foraging (Hölldober & Wilson, 1990; Vittori et al., 2006) by reinforcing shorter paths (Deneubourg, Aron, Goss, & Pasteels, 1990) and marking high‐quality food (Jackson & Chaline, 2007). To ensure that this environmental information is available for a long period of time, slow‐evaporating chemicals are used, while short‐lived chemicals serve to enhance the saliency of particular sites (Jackson & Chaline, 2007). Patterns of contact can also serve as a sign: When a “passive” harvester ant, Pogonomyrmex barbatus, at the nest side has had a certain number of interactions with successful foragers returning to the nest, it becomes an “active” forager as well (Greene & Gordon, 2007). This positive feedback loop enables flexible and efficient adaptation of the number of foragers to a given workload.
Signaling also plays an important role in human coordination. When performing tasks that involve a physical coupling between coactors (e.g., pulling on different sides of a pendulum to make it move at certain frequencies) people increase movement forces and produce more overlapping forces, thereby enhancing haptic information flow between them (van der Wel, Knoblich, & Sebanz, 2011). Musicians rely on visual signaling like exaggerating head or arm movements in order to enhance acuity of temporal coordination (Goebl & Palmer, 2009). Recent studies have shown that people modulate their hand movements to indicate which part of an object they are going to grasp (Sacheli, Tidoni, Pavone, Aglioti, & Candidi, 2013). This indicates that instrumental actions can be modified to serve as communicative signals (Csibra & Gergely, 2009; Pezzulo & Dindo, 2011; Pezzulo, Donnarumma, & Dindo, 2013).
Learning about contingencies between one’s own and others’ actions and following heuristics can also facilitate coordination. For instance, when capuchin monkeys, Cebus apella, were able to gain access to food by simultaneously pulling on bars with another agent, they learned the contingency between the presence of another agent and success, and they adjusted their pulling behavior accordingly (Mendres & de Waal, 2000). Similarly, chimpanzees learn which of their previous collaborators was efficient and choose this individual for further collaborations (Melis, Hare, & Tomasello, 2006). Furthermore, coordination can be made more precise, temporally and spatially, when the individuals in a group acquire coordination rules. Group hunts, for instance, require that individuals learn to spatially align with at least one group member and adjust the starting point and/or speed of the attack on the basis of the other hunters’ behavior. Although cooperative hunts often appear to involve strategic planning, simple algorithms such as “always move where you can keep prey in between you” may underlie this behavior (Holekamp, Boydston, & Smale, 2000). Thus, learning to quickly respond to others’ moves so as to minimize distance to the prey and to keep equidistant from cohunters is at the core of most group hunts, even when they are extremely demanding. Chimpanzees, Pan troglodytes, when hunting small monkeys who move fast and unpredictably in all three spatial dimensions, learn how to constantly monitor and anticipate the behavior of both the prey and their cohunters (Boesch & Boesch, 1989; Boesch, 2003). When joint actions require precise temporal coordination, humans have been shown to apply simple heuristics such as speeding up their movements in order to enhance predictability. For instance, pairs of participants were requested to time their responses to stimuli so that their actions were performed simultaneously. By increasing response speed as much as possible, participants reduced the variability of their response times and increased the predictability of their actions (Vesper, van der Wel, Knoblich, & Sebanz, 2011). It has recently been shown that macaque monkeys, Macaca mulatta, also reduce the variability of their actions in a joint coordination task (Visco‐Comandini et al., 2015).
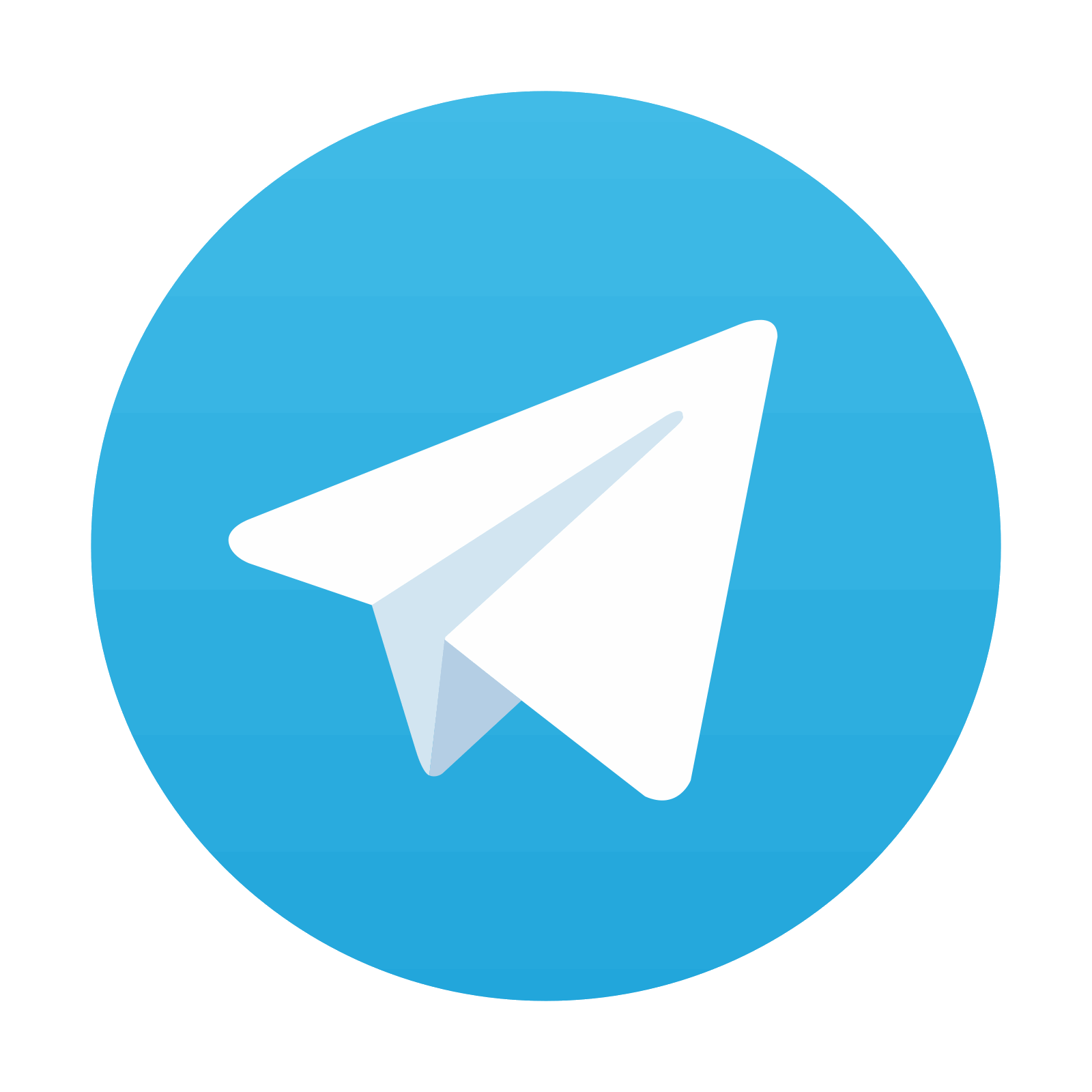
Stay updated, free articles. Join our Telegram channel
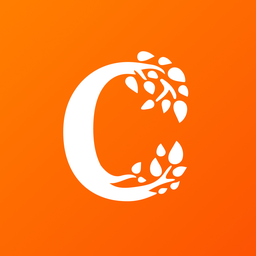
Full access? Get Clinical Tree
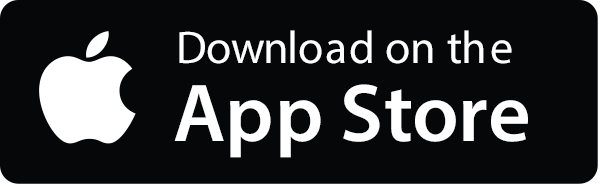
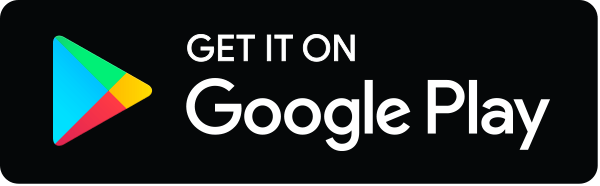