Neurotransmission—Evolving Systems
Michel Anctil
10.1 Introduction
Connecting with others is a deeply entrenched characteristic of our social self and we routinely see this played out in the animal world. In the living world at large we witness the trappings of connectivity in the form of signals carrying meanings that are not always clear to us. At the cellular level we have become accustomed to linking the emission of a chemical signal with a cascade of reactions. We regard this as the modus operandi of signaling systems, the linchpin of cellular communication.
Trams (1981) theorized that signaling systems evolved in five steps of increasing complexity: (1) basic “chemoception” first present in unicellular organisms; (2) open‐loop communication, in which a molecule is widely broadcast and becomes recognized at a distance by an individual, as in chemotaxis; (3) closed‐loop communication, in which two‐way chemical information transfer occurs between cells of a multicellular organism; (4) communications networks in which signaling is diversified through multiple molecular channels allowing more complex excitation–response processes; and (5) symbolic logic exchanges, in which hierarchically layered neural networks mediate aggression, courtship, and other complex behaviors. In this scheme, the emergence of neurons and neurotransmission fits in the third step.
The activity of a cell’s signaling systems can be controlled by a number of external factors. In neurotransmission, the transfer of a message from a neuron to another neuron, or from a neuron to an effector cell, is a controlling factor that can activate or modulate the ongoing activity of a signaling pathway in the post‐junctional cell. Constructing a network of such cells is considered the most efficient way of managing the interaction of a multicellular organism with its environment. While nonneuronal cells are able to communicate with each other and to transmit information, neurons do these things better owing to two key features. First, they make specialized contacts (synapses) with each other or with other cells that ensure speedy transmission. Second, convergence or divergence of synapses to and from neurons leads to integration of multiple signals and improves the sophistication of final response outputs.
Early research on the nervous system focused, not surprisingly, on the mammalian brain or on vertebrate models. Although Ramon y Cajal and other pioneers identified contacts between neurons in histological preparations, experimentalists were hard at work to give substance to the concept of chemical neurotransmission. In spite of accumulating evidence that neurotransmitters are released by nerves and bind to receptors to effect responses in postsynaptic cells, some researchers posed stiff resistance to the concept and proposed instead that neurotransmission occurs by current flowing from one neuron to another. With the advent of electron microscopy and more sophisticated electrophysiological techniques, the existence of both chemical and electrical synapses was established and accepted. The roles of exocytosis in chemical synapses and of gap junctions in electrical synapses became basic to our understanding of neurotransmission at the cellular level.
Just as neuroscientists became comfortable with the notion of chemical synapses functioning with a limited number of small neurotransmitters binding each to a class of specific postsynaptic receptors, the discipline of comparative neurobiology emerged and forced a revision of the paradigm. Similarly, the notion of neurotransmitters and hormones operating at two separate, mutually exclusive levels was belied by the discoveries of comparative endocrinology. That neurons can synthesize and release hormones, thereby bridging the nervous and endocrine systems, was discovered thanks to pioneering investigations by Ernst and Berta Scharrer on fish and insects in the 1920s and 1930s (Sawin, 2003). These studies led to the identification of similar neurosecretory cells in the mammalian hypothalamo‐hypophyseal complex and to experimental evidence of neurohormones accumulating in nerve endings and released into the circulatory system (Bargmann & Scharrer, 1951).
From the discovery that many neural secretions are peptides to the realization that these same peptides directly influence neurons (the concept of neuropeptides) there passed only a short time, spanning the late 1960s and early 1970s (de Wied, 1984; Strand, 2007). The techniques developed to identify the first peptides acting as neurohormones (for example, high‐performance liquid chromatography coupled with radioimmunoassay) were also applied to identify peptides in the invertebrate and vertebrate CNS (Scharrer, 1990). As the realization sank in that peptide families could be found in a variety of neurons throughout the nervous system, evidence emerged that these peptides were released via similar mechanisms to classical neurotransmitters. On the postsynaptic side, it became increasingly clear that the diversity of neuropeptide receptors surpassed that of the neuropeptides themselves. However, the functional role of neuropeptides in neurotransmission has proved difficult to pinpoint. While a modulatory role as cotransmitter was demonstrated for neuropeptides in several studies, their involvement as bona fide frontline neurotransmitters cannot be dispelled, as we shall see below.
The concept of co‐transmission originated from observations of co‐localization of ATP with norepinephrine in sympathetic neurons (Burnstock, 1976). Soon after, numerous studies documented the co‐release of transmitters, usually a neuropeptide with a classical neurotransmitter or another peptide, and much of what we know of the functional implications of co‐transmission came from studies on invertebrate experimental models (Kupfermann, 1991). It is now conceivable that the majority of synapses in any nervous system are multitransmitters and it has become apparent that synapses that involve two classical neurotransmitters but no neuropeptide are much less frequent in both vertebrates and invertebrates (Miller, 2009; Trudeau, 2004).
Comparative neurobiology provided relevant animal models amongst invertebrates and lower vertebrates (providing large tractable neurons) that helped illuminate fundamental features of neurotransmission at the cellular level. But comparative studies are also critical to efforts aimed at tracing the origin and evolution of neurotransmission and, more specifically, transmitters. The bulk of data accumulated so far on transmitter systems in a variety of phyla was obtained using the tools of biochemical analysis and immunohistochemistry combined with electrophysiological monitoring techniques that proved successful in assessing the range of transmitters and receptors available to support the behavioral repertoire proper to each animal grade. With the advent of molecular tools and the recent opportunities for genomic analysis, a new era of evolutionary neuroscience is unleashed, opening up the field for more targeted gene expression studies and phylogenetic analyses of neurotransmitter/receptor systems.
These developments led to the views on the evolution of neurotransmitters that serve as the basis for the following narrative. Figure 10.1 summarizes some hypothetical phylogenetic steps that led to electrical and chemical neurotransmission.
10.2 Unicellulars and Neurotransmitters: The Concept of Biomediators
Our view of neurotransmitters as unique to neurons was challenged at about the same time as our view of hormones as unique to endocrine tissues. In this case, the leadership did not come from the comparative neurobiology and endocrinology community, but from researchers in the medical field. It started with reports of the detection of insulin‐related peptides and conventional neurotransmitters in fungi and the ciliated protozoan Tetrahymena in the early 1980s (Le Roith, Shiloach, Roth, & Lesniak, 1980; Roth et al., 1985).
Although these findings threatened to alter the consensual view of what constitutes a neurotransmitter, evidence of autocrine or paracrine signaling pathways for the putative transmitters was often absent or sketchy. Sometimes the case for the putative transmitter was merely its biochemical detection or its effect on some activity by the unicellular. It was seldom clear whether the substance was released by the cell as a step in a signaling pathway or as an inactive by‐product of some separate activity. Also, the putative transmitters must be released outside the cell for signaling to occur. Does that involve release mechanisms proper to metazoan synapses, such as exocytosis? Exocytosis of dense‐cored vesicles was visualized in detail in Paramecium, in which vesicle docking and membrane fusion appear to involve proteins similar to those associated with synapses of multicellular animals (Plattner & Kissmehl, 2003). However, key proteins such as SNARE and V0 components have yet to be detected, so the jury is still out as to how strongly the release mechanisms in paramecia resemble the presynaptic machinery of metazoans.
More recent evidence supports the view that substances acting as fast neurotransmitters in many metazoan synapses are likely candidates for regulatory function in protozoan development, ciliary locomotion, and intercellular communication. This applies particularly to acetylcholine (ACh) and the amino acids glutamate and GABA. Acetylcholine and its biosynthetizing enzyme, choline acetyltransferase (ChAT), were detected in the ciliate Paramecium (Horiushi et al., 2003; Kawashima et al., 2007). Expression levels of ChAT were high in mature individuals but absent in immature individuals; blocking acetylcholinesterase activity led to a significant reduction of cell pairing during mating (Delmonte Corrado et al., 2001; Trielli, Politi, Falugi, & Delmonte Corrado, 1997). Thus, the case is strong for a role of ACh in Paramecium conjugation.
Although glutamate is not known to accumulate in ciliates, highly specific L‐glutamate binding sites were reported on the cilia membranes of Paramecium (Preston & Usherwood, 1988a), and L‐glutamate was found to induce a transient membrane hyperpolarization and to act as a chemoattractant for swimming paramecia (Preston & Usherwood, 1988b). The reason for their attractiveness to ciliates is that release of glutamate by bacteria serves as a signal that food is at hand (Van Houten, 1978). In addition, the action of glutamate is mediated by a fast increase in intracellular cAMP (Yang, Braun, Plattner, Purvee, & Van Houten, 1997), suggesting that the hallmarks of glutamatergic signaling are present and functioning in paramecia. More recently, a candidate gene for a NMDA‐like receptor was identified when its down‐regulation by RNA interference caused the loss of the chemoresponse of paramecia to L‐glutamate (Valentine, Yano, & Van Houten, 2008). Furthermore, searching the genome survey sequence (GSS) and EST transcripts of another ciliate, Tetrahymena, for receptor proteins yielded a putative gene sequence matching NMDA receptors (Orias, 2002).
In contrast to glutamate, GABA was reported to be present in paramecia and released in the external medium by KCl‐induced depolarization (Delmonte Corrado et al., 2002; Ramoino et al., 2003). Pharmacological experiments indicated that GABA modulates swimming in paramecia through two signaling pathways. One pathway appears to promote forward swimming through GABA‐A receptors and Ca2+ entry (Bucci, Ramoino, Diaspro, & Usai, 2005) whereas the other pathway promotes ciliary reversal and backward swimming through GABA‐B receptors, G protein activation and inhibition of Ca2+ entry (Ramoino et al., 2003). Although no attempt was made to back these studies with evidence of GABA binding sites on the ciliate’s membranes, the evidence for the presence of conventional GABAergic pathways in ciliates is strong and supported by reading matchups to GABA receptors in a GSS of the ciliate Tetrahymena (Orias, 2002).
With these and other reports of the presence of ACh and biogenic amines in microorganisms and plants (see Freestone & Lyte, 2008; Murch, 2006 for reviews), it becomes more and more tempting to conclude that classical neurotransmitters should be considered as multifunctional substances universally involved in physiological processes in all living organisms (see also Chapter 4). Roshchina (2010) proposed that, in view of this ubiquity, calling these substances “biomediators” instead of neurotransmitters or neurohormones is more accurately descriptive of their status in biological signaling. She advances the hypothesis that such biomediators were first involved as participants in chemotaxis through their secretion in the environment as attractants or repellents to other cells searching for food or mating partner. This would have served as the basis for cell‐to‐cell communication in a fundamental ecological community or ecosystem. Because chemotaxis involves a ligand‐receptor relationship, this type of basic cellular signaling would have more likely carried over without much ado in multicellular organisms, by simple inheritance from unicellulars.
10.3 Sponges: The Trappings of Neurotransmission without the Neurons
Although there is disagreement over the phylogenetic position of sponges (Edgecombe et al., 2011), phylogenomic studies (Philippe et al., 2009) and the recent sequencing of the genome of a sponge from the Great Barrier Reef (Srivastava et al., 2010) support the view that sponges are a sister group to the Eumetazoa (Cnidaria, Placozoa, and Bilateria). Therefore, sponges stand at a pivotal position in the evolution of cell‐to‐cell signaling in multicellulars, and especially concerning neurotransmission. However, sponges lack neurons and, as a result, share with unicellulars the ambiguity of determining how “neuroid,” to use Parker’s (1919) expression, their modes of cell‐to‐cell communication are. Recent discussions have revolved around the hypothesis that sponges, being able to detect external stimuli and produce coordinated effector responses, possess sensory cells and semi‐specialized epithelial cells (myocytes) capable of transmitting chemical signals (Nickel, 2010; Renard et al., 2009). A similar scenario holds for the small, obscure phylum Placozoa (see Figure 10.1), but, as little is known about transmitters in the group except for evidence of aminergic‐like elements in the genome (Srivastava et al., 2008), the focus of this section will remain on sponges.

Figure 10.1 Proposed evolutionary steps for the emergence of transmitter systems. The phylogenetic relationships are based on the tree proposed by Moroz et al. (2014). The two nodes represent independently evolved traits in Ctenophore and the Cnidaria/Bilateria lineages (homoplasy).
For a start, do sponges, as multicellular assemblies, possess the building blocks of neurotransmission? The answer appears to be a qualified yes. The sequenced genome of the demosponge Amphimedon queenslandica reveals a large number of genes involved in the assembly and functioning of both pre‐ and postsynaptic elements in eumetazoans (Srivastava et al., 2010). These include scaffolding molecules that manage the deployment of synaptic vesicles, calcium channels, and signaling machinery on the presynaptic side, and proteins (ion channels and receptors) on the postsynaptic side. In addition, A. queenslandica possesses genes that encode proteins involved in the regulation of synaptic vesicle exocytosis by calcium influx (synaptophysin, synaptotagmin, SV2). Calcium influx appears to be implicated in sponges because it was demonstrated that contractile responses to transmitters cannot be propagated (presumably from cell to cell) in calcium‐free media (Elliott & Leys, 2010). However, the lack of a homolog of RIMS, a scaffold for the facilitation of synaptic vesicle fusion in exocytosis, casts doubt on the potential ability of sponges to effect synapse‐like transmission. As for the other sequences, a cautionary note is in order as to the reliability of the relatively superficial annotations associated with the disclosure of genome sequences. It has been the experience of this author that closer, manual inspections of the sequences sometimes reveal erroneous annotations in the genome, as hits on a protein family do not necessarily convert into a bona fide match (Anctil, 2009). Given this caveat, some of the claims for the presence of synapse genes in this sponge may turn out to be invalid after a more thorough analysis. This can apply to other gene families discussed further below.
If expression of synaptic proteins remains to be demonstrated in sponges, are classical transmitters such as those found in unicellulars present in sponges and processed through some synaptic‐like machinery? Early histochemical investigations reported the presence of acetylcholinesterases and biogenic amines in sponges (Lentz, 1966), but the poor reliability of the techniques used and the lack of supporting evidence for the involvement of these substances in signal processing have cast doubt on the claims (Mackie, 1990). However, recent studies have provided convincing physiological evidence for a role of the amino acid transmitters glutamate and GABA in coordinating behavior in sponges. Two laboratories independently reported propagated contractions in sponges induced by glutamate (Elliott & Leys, 2010; Ellwanger & Nickel, 2007) and their inhibition by GABA (Elliott & Leys, 2010), in keeping with the traditional opposite actions of these transmitters in higher animals. In addition, Elliott and Leys detected by HPLC relatively large amounts of glutamate in sponge tissues, although no glutamate or GABA released in the medium could be detected after KCl‐induced depolarization of those tissues. A third transmitter, nitric oxide (NO), was found to induce contractions and modulate the amplitude and frequency of endogenous rhythmic contraction waves (Ellwanger & Nickel, 2006). Nitric oxide synthase activity was visualized in cells of the osculum and in pinacocytes, considered to be the substrates for coordinated responses in the demosponge Ephydatia muelleri (Elliott & Leys, 2010). A common target in NO signaling pathways of eumetazoans is cyclic guanosine monophosphate (cGMP), and NO was shown to increase cGMP production in the osculum and pinacoderm of E. muelleri (Elliott & Leys, 2010).
These findings are supported by (1) the cloning in the sponge Geodia cydonium of a metabotropic glutamate receptor with apparent mixed selectivity for glutamate and GABA (Perovic, Krasko, Prokic, Müller, & Müller, 1999) and (2) the presence of sequences matching metabotropic glutamate receptors and the PDZ domain of a glutamate receptor interacting protein in the A. queenslandica genome (Srivastava et al., 2010). In contrast, no GABA receptor sequence was identified, which begs the possibility of a nonspecific action by GABA on sponge contractility, a suspicion strengthened by the conflicting results of Ellwanger and Nickel (2007) and Elliott and Leys (2010) with GABA. On the other hand, the presence of a NOS sequence in the genome is consonant with the nitrergic signaling pathway uncovered by Elliott and Leys (2010). The genome also revealed sequences apparently related to dopamine and serotonin receptors. While it is premature to label these sequences as such, in view of the difficulty of finding close orthologues of specific biogenic amine receptor subfamilies even in cnidarians (Bouchard, Ribeiro, Dubé, & Anctil, 2003; Bouchard, Ribeiro, Dubé, Demers, & Anctil, 2004), a report of serotonin immunoreactivity in cells of a sponge larva and settled juvenile (Weyrer, Rutzler, & Rieger, 1999) encourages further inquiry.
In addition to all these candidate transmitters, many sequences related to neuropeptide production and secretion were identified in the genome of A. queenslandica (Srivastava et al., 2010). These include peptidases that cleave precursor chains to produce active peptides (proprotein convertases, an aminopeptidase, a carboxypeptidase, and cathepsins), a gene for post‐translational C‐terminal amidation (PAM) and another for N‐terminal pyrolation, and finally two genes encoding proteins involved in the calcium‐dependent secretion of neuropeptides. Despite this complex machinery, no neuropeptide precursor homologue was detected in the sponge genome. As Baggerman, Liu, Wets, and Schoofs (2005) have noted, it is notoriously difficult to hunt for neuropeptide precursor proteins in genomes when there is no biochemically isolated peptide or cloned sequences at hand, as is the case with sponges. Even when some are available, as in the sea anemone Nematostella vectensis, locating the homologues in the genome is a painstaking process (Anctil, 2009). However, the presence in the sponge genome of G‐protein‐coupled receptors (GPCR) belonging to the LGR/hormone, opsin/prostanoid and gonadotropin‐releasing hormone/neurotensin/somatostatin receptor families should serve to encourage efforts to uncover neuropeptide‐like transmitters in the sponge genome. A recent report of sponge larval settlement triggered by the cnidarian neuropeptide GLWamide (Whalan, Webster, & Negri, 2012) is a step in the right direction.
From the evidence reviewed above, it is clear that sponges use chemical substances known to act as neurotransmitters in neurons of eumetazoans in order to coordinate their behavior, and that they do so through paracrine channels of transmission in the absence of neurons (Elliott & Leys, 2010; Nickel, 2010). Their “management style” of signal transmission appears to be a mere upgrade from that of unicellulars like paramecia. However, the sponge’s tissue integration, as opposed to a loose community of unicellulars, ensures that cells are more proximal to each other and that relatively tight coordination of activities by chemical transmission can be achieved through a chain of epithelial cells. Leys and Riesgo (2012) have recently argued that true functional epithelia appeared first in sponges. This is where the innovation in transmission stands—not in the chemical identity of the transmitters, which is carried over from the unicellulars and which may include acetylcholine, glutamate, and GABA.
10.4 Cnidarians: Neurotransmission Enters the Stage
Recent analyses of basal metazoan relationships based on molecular phylogeny have been mired in controversy. One such analysis claiming to use more appropriate methods and larger sampling of genes proposes that Ctenophora and Cnidaria, which share the possession of nervous systems and muscle cells, are sister groups in a clade (the revived Coelenterata) which branched early from the bilaterian line (Philippe et al., 2009). On that basis nervous systems would have originated in a common ancestor of these groups. In addition, it was previously assumed that extant ctenophores are more derived forms than cnidarians (Bridge, Cunningham, Schierwater, DeSalle, & Buss, 1992).
However, the recent sequencing of the genome of the ctenophore Pleurobrachia bachei has allowed new insights into the set of neural genes of this phylum that challenge any view of cnidarians and ctenophores sharing close affiliations (Moroz et al., 2014). Quite to the contrary, this new analysis leads to the surprising conclusion that ctenophores constitute “the most basal animal lineage” and that sponges are “the sister taxon to remaining metazoans” (Moroz et al., 2014, p. 109). Ctenophores lack many of the neural genes associated with cnidarians and bilateral animals, suggesting that neurons arose independently in ctenophores and in the cnidarian/bilaterian lineage. What is particularly relevant to this chapter is the lack in ctenophores of nonpeptidergic neurotransmitter systems with the possible exception of glutamate, thus reinforcing the notion that these are cnidarian/bilaterian innovations. Because the implication of the study is that cnidarians constitute the sister clade of bilateral animals and that the nervous system of ctenophores was an evolutionary dead end, in the following account cnidarians will serve as representatives of the basal nervous system shared with the lineage of the bilaterians. All extant cnidarian species examined so far possess neurons organized in assemblies ranging from diffuse, two‐dimensional nerve nets to condensed, ganglion‐like “nervous centers” (Mackie, 2004; Satterlie, 2011). For more on how they got there—presumably from a basal ancestor with a level of tissue organization between sponge and cnidarian grades—refer to Chapters 5 and 6 of this volume. This initial emergence of neurons in Metazoa set the stage for a remodeling of cell‐to‐cell signal transmission through the coemergence of specialized contact zones, known as synapses.
Electrical synapses—gap junctions—make their first appearance in Cnidaria and Ctenophora, where they link epithelial cells or neurons to propagate behavioral control signals (Mackie, 2004; Mackie & Passano, 1968; Spencer, 1981; Spencer & Satterlie, 1980). While this mode of signal transmission is important as an adjunct to chemical transmission, it has only been morphologically and functionally demonstrated in one cnidarian class, Hydrozoa (but see Germain & Anctil, 1996; Mire, Nasse, & Venable‐Thibodeaux, 2000).
Contrary to sponges, where traces of synaptic machinery must be sniffed out by genomic analysis, in cnidarians the synapses observed by electron microscopy possess most of the familiar features seen in invertebrate and vertebrate synapses (see Westfall, 1970, 1996 for review). Due to their epithelial position, sensory cells tend to make unidirectional synaptic contacts with neurons of the nerve nets (Holtmann & Thurm, 2001), and cnidarian neuromuscular synapses are likewise unidirectional (Spencer, 1982). But transmission is nonpolarized in the sheet‐like nerve nets, with many bidirectional, symmetrical synapses found between neurons. Based on reconstruction of serial sections from the jellyfish Cyanea capillata, these early versions of synapses are characterized by a single layer of a few large synaptic vesicles abutting the terminal membrane, an arrangement mirrored on the opposite side of the terminal (Anderson & Grünert, 1988). Electrophysiological analysis of these jellyfish synapses revealed that postsynaptic potentials are indeed generated alternately in the opposite neuron, so that each synaptic partner can play a presynaptic role at one moment and a postsynaptic one just a few milliseconds later (Anderson, 1985). These synapses display unusually high depolarization thresholds for transmission to avoid continuous “babbling” between the two partners and loss of meaningful signal transmission.
Conventional electrophysiological approaches, such as those used by Anderson (1985), allow chemical transmission only to be indirectly monitored from the postsynaptic side. To directly record presynaptic events such as exocytosis in real time, amperometry with carbon‐fiber microelectrodes was used to record currents generated by electro‐oxidizable monoamines released from isolated neurons of the sea pansy Renilla koellikeri (Gillis & Anctil, 2001). Depolarization‐evoked transmitter release displayed the fast kinetics and calcium sensitivity typical of exocytotic events in neurons of higher invertebrates (Bruns & Jahn, 1995; Chen & Ewing, 1995; Chen, Gutman, Zerby, & Ewing, 1996). Many larger exocytotic events appeared as multiples of a subunit spike, and therefore suggested a quantal mode of secretion as classically defined for mammalian neuromuscular synapses by Boyd and Martin (1956), but the skewed distribution of spikes in favour of the lowest (subunit) amplitude contradicted the classical model (Gillis & Anctil, 2001).
With the hindsight of more recent data from mammalian cell models, it is likely that the fewer, larger spikes (≥5 pA) recorded in sea pansy neurons represent the complete emptying of a vesicle (all‐or‐none exocytosis) whereas the higher frequency spikes of small amplitude (<5 pA) reflect the partial discharge of transmitter through the fusion pore (kiss‐and‐run exocytosis). These mechanisms have been observed in chromaffin cells (Henkel, Kang, & Kornhuber, 2001) which, like the sea pansy, contain catecholamines stored in large dense‐cored vesicles; they are also seen in rat dopaminergic neurons (Staal, Mosharov, & Sulzer, 2004). Even the strong bursts of fusion pore flickers seen in chromaffin cells, an indicator of secretion “hot spots” (Henkel et al., 2001), are present in the majority of the recorded monoaminergic neurons of the sea pansy (Gillis & Anctil, 2001). Staal et al. (2004) proposed that kiss‐and‐run exocytosis increases the longevity of a synaptic vesicle, a distinct advantage in synapses where synaptic vesicles are low in number such as in cnidarian synapses. It is clear from these observations that sophisticated delivery of transmitter at the synapse was an early step of neuronal evolution that underwent little, if any, change up to mammals.
With synapses fully operational in Cnidaria, we may ask to what extent substances reach their full potential as neurotransmitters. The question is legitimate, insofar as declaring a candidate to be a neurotransmitter requires that the substance meets stringent criteria: synthesis in presynaptic neuron, presence in presynaptic terminal vesicles, release upon stimulation (depolarization), binding to specific postsynaptic receptors and production of a fast, reversible response in the target cell. While an impressive array of transmitters satisfy some of the criteria in Cnidaria, as documented in the comprehensive review by Kass‐Simon and Pierobon (2007), very few have been shown to meet all of them, likely due in part to difficulties in finding tractable experimental models at the cellular level. Genomic approaches, such as the survey of sequences matching those of transmitter systems in the genome of the starlet sea anemone N. vectensis (Anctil, 2009), may help to focus efforts on cloning and expressing candidate genes, thereby establishing neurotransmitters on more solid ground, but this will require substantial investments from many researchers.
Let us first examine which of the candidate transmitters have the best case for a neurotransmitter role in Cnidaria. Three candidate classes include peptides, amines, and amino acids. The RFamide‐related peptides (RFaPs), a major neuropeptide family, are strong contenders. Not only are they present exclusively in neurons of all the species examined so far from all cnidarian classes, but neurons containing them form nerve nets and appear to be more numerous than any other class of chemically identified neurons. The precursors of these peptides contain multiple copies of the peptide (up to 36 copies in the sea pansy), thus leading to high concentrations of the bioactive peptide in the terminals. One of them, Antho‐RFamide, was localized by immuno‐electron microscopy in synaptic vesicles of hydra and sea anemones (Koizumi, Wilson, Grimmelikhuijzen, & Westfall, 1989; Westfall & Grimmelikhuijzen, 1993), but synaptic release has not been investigated. In addition, it was demonstrated that Hydra RFamides were ligands for a Hydra peptide‐gated ion channel (Golubovic et al., 2007), thus qualifying them as fast neurotransmitters, a highly unusual role for neuropeptides which, as a rule, bind to metabotropic receptors, usually rhodopsin‐like G‐protein‐coupled receptors (GPCRs). The only other report of a neuropeptide‐gated ion channel relates to a snail (Cottrell, 1997; Cottrell, Jeziorski, & Green, 2001) and also involves a RFaP, thus suggesting that these peculiar ionotropic receptors are evolutionarily ancient. There is physiological evidence for a role of RFaPs as neuromuscular transmitters in cnidarians (Anctil & Grimmelikhuijzen, 1989; McFarlane, Graf, & Grimmelikhuijzen, 1987), but these actions appear to be mediated by as‐yet unidentified metabotropic receptors.
Biogenic amines are the next best contenders. There are numerous reports of the presence and actions of biogenic amines in Cnidaria (see Kass‐Simon & Pierobon, 2007 for review), but none provide multiple converging lines of evidence except in one anthozoan, the sea pansy R. koellikeri. The enzyme machinery for the synthesis of dopamine and norepinephrine appears to be present and expressed in neurons (Anctil, Hurtubise, & Gillis, 2002; Pani & Anctil, 1994) and even in nerve terminals of a sea anemone (Westfall, Elliott, MohanKumar, & Carlin, 2000), although genomic evidence from the starlet sea anemone suggests that the enzymatic pathways differ from those of higher invertebrates and vertebrates (Anctil, 2009). Serotonin and norepinephrine immunoreactivities were detected in sea pansy neurons (Umbriaco, Anctil, Descarries, 1990; Pani, Anctil, & Umbriaco, 1995), but only in a sea anemone has serotonin been localized in synaptic vesicles (Westfall et al., 2000). Norepinephrine was released from sea pansy tissues by KCl‐induced depolarization in a calcium‐dependent manner (Pani et al., 1995) and neuronal catecholamine release was confirmed at the cellular level by Gillis and Anctil (2000) as mentioned earlier. Adrenergic and serotonergic receptors were characterized by analysis of membrane binding sites in the sea pansy (Awad & Anctil, 1993; Hajj‐Ali & Anctil, 1997) in relation to their roles in bioluminescence control and modulation of peristaltic contractions in this species (Anctil, 1989; Anctil, Boulay, & LaRivière, 1982). Two receptors for biogenic amines were also cloned from the sea pansy (Bouchard et al., 2003, 2004), but none correspond to those characterized by membrane binding and in fact their ligand is unknown at this point. Numerous orthologues of biogenic amine receptors, transporters for biogenic amine re‐uptake and aminergic vesicular transporters were also found in the genome of the sea anemone N. vectensis (Anctil, 2009).
The third contenders are the amino acids taurine/β‐alanine. In a search for the fast transmitter involved in the bidirectional synapses of the previously‐mentioned jellyfish nerve net (Anderson, 1985), taurine immunoreactivity was localized in motor nerve net neurons (Carlberg, Alfredsson, Nielsen, & Anderson, 1995). Anderson and Trapido‐Rosenthal (2009) have since reported that both taurine and β‐alanine induce depolarizations and conductance changes in nerve‐net neurons that are consistent with the excitatory postsynaptic potentials recorded during synaptic activity. They also found that both amino acids were released by depolarizing nerve‐net neurons, but so were other amino acids, and the calcium sensitivity of release could not be demonstrated (Anderson & Trapido‐Rosenthal, 2009). In addition, it is unknown if these amino acids are stored in synaptic vesicles, and nothing is known about their receptors. While this is the best existing model to identify a fast transmitter at an identified synapse, there is still a lot of work ahead to confirm these amino acids as cnidarian neurotransmitters.
Other amino acids were enlisted as candidate transmitters in Cnidaria. Ionotropic receptors for glutamate, GABA and glycine were characterized by pharmacological analysis of binding sites from membranes of Hydra vulgaris (Concas et al., 1998; Grosvenor, Bellis, Kass‐Simon, & Rhoads, 1992; Pierobon et al., 1995, 2001, 2004). Receptors for all three putative transmitters are represented in transcripts of the genome of N. vectensis, including metabotropic receptors (Anctil, 2009). Vesicular transporters for glutamate and for GABA were also spotted in the genome, suggesting that these amino acids have the potential to be stored in synaptic vesicles. There are a number of reports pointing to a role for these transmitters in the control of nematocyst discharge (Kass‐Simon & Scappaticci, 2004), pacemaker activity in motor systems (Kass‐Simon, Pannaccione, & Pierobon, 2003; Ruggieri, Pierobon, & Kass‐Simon, 2004), and the feeding response (Pierobon et al., 1995, 2001, 2004).
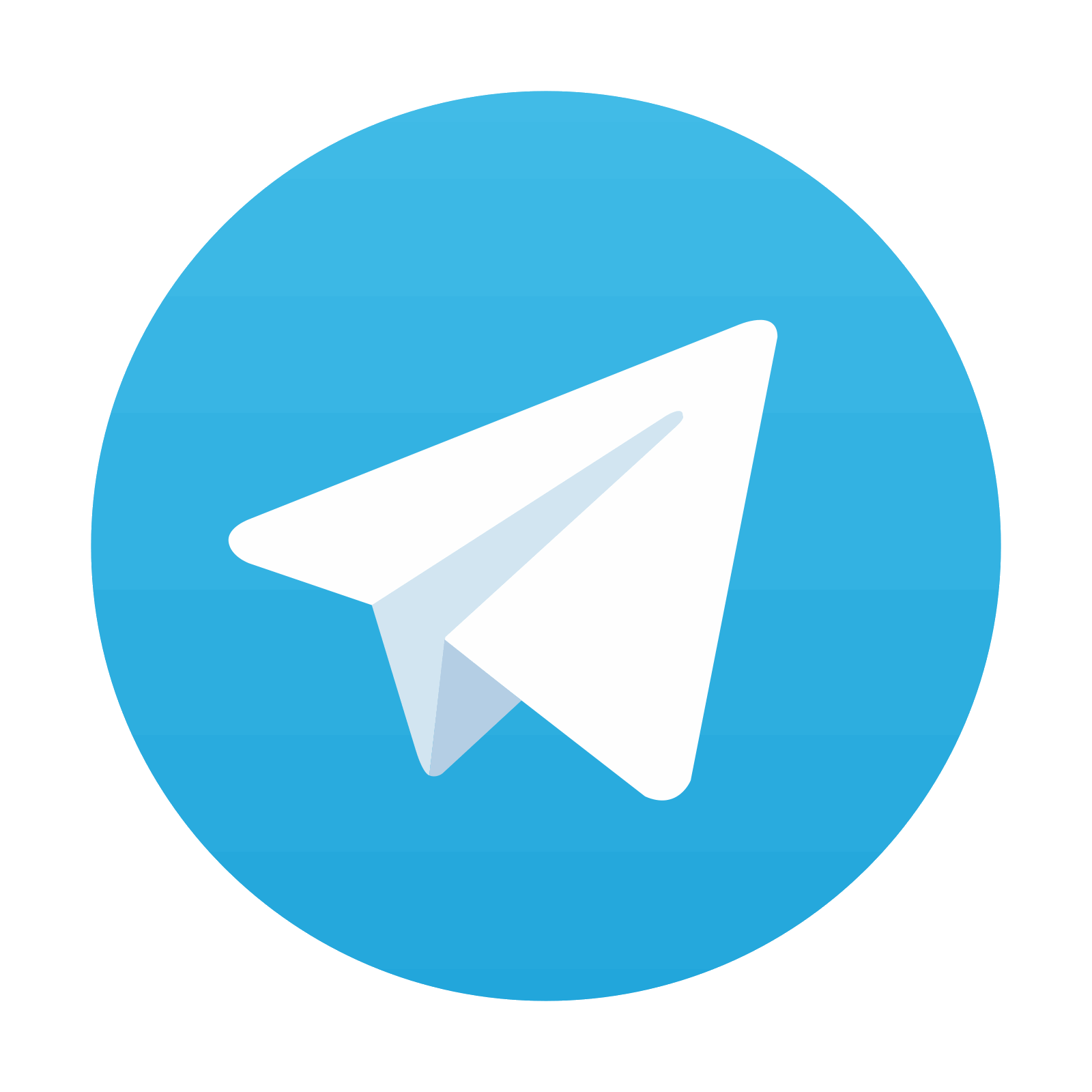
Stay updated, free articles. Join our Telegram channel
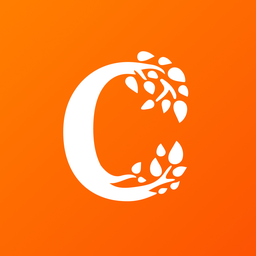
Full access? Get Clinical Tree
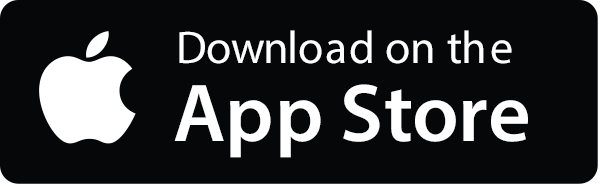
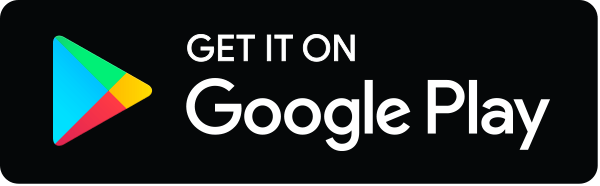