Fig. 7.1
Differentiation fate of transplanted human fetal-derived NSCs and their effects on behavioral recovery after stroke. a Human fetal-derived NSCs (human nuclear antigen (HuNu)-positive cells, red) survive and migrate towards the stroke lesion at 5 weeks post-IC transplantation in athymic nude rats subjected to cortical ischemic stroke. Representative photomicrograph of human fetal-derived NSCs in the peri-infarct region. Gray-shaded area indicates the lesion. b Differentiation profile of human fetal-derived NSCs at 5 weeks post transplant. c Confocal images of differentiation markers colocalizing with HuNu in the peri-infarct area. d Rats treated with human fetal-derived NSCs have significantly improved functional recovery compared to vehicle-treated controls in three out of four behavior tests; * P < 0.05, ** P < 0.01; n = 12 per group, except cylinder test, n = 6. Scale bars: A = 50 µm, C = 10 µm. GFAP glial fibrillary acidic protein, TuJ1 neuronal class III β-tubulin, Tx transplantation. (This figure was reprinted with the publisher’s permission from [29])
Embryonic-Derived Neural Stem Cells
Following the establishment of cultured human embryonic stem cell lines [26], several groups, including ours, have developed methods to generate NSCs from human or primate embryonic stem cells and have demonstrated their ability to enhance functional recovery following IC administration in preclinical models of stroke [21, 33–37]. Interestingly, in a study comparing IC delivery of embryonic- and adult-derived NSCs into stroke-injured rats, embryonic-derived NSCs exhibited substantially higher survival that was correlated with a decreased host immune response [38]. These promising preclinical results, along with the capacity for potentially limitless scale-up of the starting embryonic stem cell population, make embryonic-derived NSCs an attractive cell therapy candidate for stroke, and continued efforts towards clinical application are underway (Steinberg/Carmichael: California Institute for Regenerative Medicine (CIRM) Disease Team Grant DR1 01480) [39, 40].
Induced Pluripotent Stem Cells
Recently, excitement has increased regarding the possibility of using adult-derived NSC therapies to treat stroke due to the emergence and continued development of induced pluripotent stem cell (iPSC) technologies [41, 42] as well as methods to differentiate iPSCs into NSCs [43]. Preclinical stroke studies have confirmed the ability of iPSC-derived NSCs to enhance stroke recovery following IC delivery [44–47], including in neonatal stroke [48], and have further shown that iPSC-derived NSCs can differentiate into multiple types of functionally active neurons following IC delivery into the stroke-injured brain [45, 46]. Similar to embryonic-derived NSCs, the potential to form tumors or ectopic tissue by iPSC-derived NSCs remains a concern, prompting development of methods to generate induced NSCs (iNSCs) or induced neuronal (iN) cells directly from somatic cells without initial reversion to a pluripotent state [49, 50]. Although iNSCs have not yet been tested for safety/efficacy in preclinical stroke models, the prospects of patient specificity and decreased tumorigenicity make the clinical attraction of this approach clear.
Overall, NSCs from multiple sources have been shown to improve recovery in preclinical stroke models, with specific benefits and drawbacks associated with each NSC type. In order to maximize the potential benefit of NSC-based approaches, it is important to understand the underlying mechanism(s) by which NSCs improve stroke outcome. While there are still many remaining questions regarding the therapeutic actions of transplanted NSCs, it is becoming increasingly clear that NSCs have the capacity to impact multiple pathways involved in brain repair and regeneration after stroke.
Mechanisms of Action for Transplanted NSCs in Stroke
Cell Replacement Versus Paracrine Signaling
While the initial notion was that transplanted stem cells would repair stroke-damaged circuits by replacing lost neurons, it soon became apparent that this was not the primary mechanism driving stem cell-induced recovery. Early evidence that alternative mechanisms of action were involved included the observations that NSC-associated functional improvements occurred prior to any detectable integration of the transplanted cells, and for those studies that reported some positive integration, the number of observed synapses between transplanted NSCs and host neurons was small, with a large proportion of engrafted cells remaining in an immature, precursor state [51, 52]. Based on these observations, several nonintegrating mechanisms of NSC efficacy were investigated. These focused largely on the idea that transplanted NSCs, through secretion of paracrine factors, enhance endogenous brain repair pathways that are activated after stroke. Significant data support this mechanism of action, yet a growing number of studies indicate that transplanted NSCs can also integrate into the host brain, thus renewing the idea that cell replacement, or at least host integration, may also be important.
Stimulation of Endogenous Brain Repair by NSC Paracrine Signaling
Trophic Support
NSCs have been shown to express trophic factors (proteins and other molecules) that promote survival, proliferation, and differentiation of host brain cells after stroke, and this has led to the general hypothesis of NSC-mediated recovery through trophic support [53]. In vitro profiling of NSCs has shown that they secrete such key factors as brain-derived neurotrophic factor (BDNF), glial-derived neurotrophic factor (GDNF), vascular endothelial growth factor (VEGF), and several others [29, 54–56]. However, relatively little is known about which trophic factors mediate efficacy in vivo, due in part to the need for improved methods to profile transplanted NSC expression/secretion in vivo, as well as the need for additional studies that selectively upregulate or suppress trophic factors within transplanted NSCs. For example, IC delivery of VEGF-transfected NSCs into stroke-injured rats resulted in higher levels of vascular repair and functional recovery compared to unmodified NSCs [57]. Further, a recent study by our group showed that in stroke-injured rats, the recovery enhancement associated with IC delivery of human NSCs could be suppressed by systemic infusion of bevacizumab (Avastin), an antibody specific for human VEGF [30], thus enabling us to tease apart the role of the NSC-secreted VEGF from that of endogenous rodent VEGF. Results of this study further indicated that recovery enhancement by NSCs coincided with increased neovascularization and blood–brain barrier integrity, along with decreased microglial/immune cell activation within the peri-infarct region, and that both of these changes could be suppressed by cotreatment with Avastin. These results highlight the concept that NSC-associated functional improvements are unlikely to occur via a single mechanism and instead may involve simultaneous modulation of multiple endogenous repair pathways.
Vascular Repair
Regeneration of the vascular system by localized activation of angiogenesis is one form of endogenous repair that is correlated with improved stroke outcome [58, 59] and has the potential for modulation by NSCs and other cell therapies. Different types of NSCs have been shown to express trophic factors that can stimulate neovascularization (angiogenesis or vasculogenesis), including VEGF, epidermal growth factor (EGF), insulin-like growth factor 1 (IGF1), and basic fibroblast growth factor (bFGF) [30, 60]. IC delivery of NSCs into stroke-injured rats was correlated with increased angiogenesis or neovascularization [30, 61], and poststroke stimulation of neovascularization by transplanted human fetal-derived NSCs could be suppressed by selective blockade of human VEGF [30]. Further evidence of VEGF as a key mediator of these effects was shown by intravenous coadministration of NSCs and recombinant VEGF [62] or IC delivery of VEGF-transfected NSCs [57] into stroke-injured rats; both approaches led to increased vascular repair and functional recovery beyond that of NSCs alone or unmodified NSCs. The trophic factors placental growth factor and angiopoietin-1 have been identified as candidate mediators of neovascularization by bone marrow-derived stromal cells [63–65], but their potential involvement in NSC-based therapies has not yet been elucidated.
Immunomodulation
The immune response after stroke involves both an acute phase that is associated with injury progression and a delayed phase that can aid subsequent repair [66]. Preclinical studies suggest that IC delivery of NSCs can modulate this response in favor of repair by reducing the number of activated microglia and macrophages that infiltrate surviving peri-infarct tissue [28, 30, 67]. Similarly, systemic delivery of NSCs has been shown to reduce infiltration of activated monocytes into the brain of stroke-injured rodents, and this was correlated with reduced levels of proinflammatory cytokines in the spleen and brain, including tumor necrosis factor, interferon gamma, and interleukin-6 [68, 69]. Additional in vitro studies have shown that NSCs can suppress T cell activation and dendritic cell maturation in a coculture setting, and that these effects are mediated in part by NSC secretion of such immunomodulatory factors as nitric oxide and prostaglandin E2 [70–72].
Brain Plasticity
Activation of brain plasticity after stroke in the form of outgrowth and remapping of neuronal projections is correlated with improved behavioral outcome, and thought to be a driving factor behind spontaneous recovery after stroke [73–75]. Based on studies from our group, IC delivery of human fetal-derived NSCs augmented several aspects of brain plasticity in stroke-injured adult rats, including remapping and increased axonal sprouting of contralesional neurons into the ischemic hemisphere and through the cortical thalamic and cortical spinal tracts, increased dendritic branching in both cortical hemispheres, and increased axonal transport [29, 30]. Similarly, IC delivery of human embryonic-derived NSCs enhanced axonal sprouting of contralesional neurons in a rodent model of neonatal hypoxia–ischemia [76]. Moreover, the plasticity-enhancing effects of fetal-derived NSCs could be recapitulated in vitro with a primary neuron coculture system, and the use of neutralizing antibodies implicated SLIT and thrombospondin 1 and 2 as NSC-secreted factors potentially driving these effects [29]. In addition, IC-transplanted human embryonic-derived NSCs were shown to promote recruitment and/or proliferation of endogenous oligodendrocytes in the ischemic hemisphere [77], as well as differentiate into oligodendrocytes themselves [33, 77, 78], thus demonstrating the potential to support or even directly participate in the myelination of newly formed circuits.
Neurogenesis
In response to a stroke injury, endogenous NSCs residing in the subventricular zone of the lateral ventricles can undergo proliferation and migrate to the lesion’s boundary, where a small proportion become mature neurons and integrate into surviving circuits [79–81]. In rats subject to striatal ischemic stroke, subsequent IC delivery of human fetal-derived NSCs was correlated with increased migration and survival of immature neurons in the infarct area [67]. However, given that the majority of recruited cells either die or remain in an immature state upon arrival to the infarct site [79, 82], the exact mechanisms by which injury- and cell therapy-induced neurogenesis might lead to improved stroke recovery still need to be resolved and may be more akin to various types of therapeutic paracrine signaling attributed to transplanted NSCs.
Integration into the Host Brain
Although an increasing number of preclinical studies suggest that transplanted NSCs improve stroke outcome by stimulating endogenous repair through paracrine actions, there are a growing number of reports that transplanted NSCs can functionally integrate into surviving host circuits, raising the question regarding the significance of this integration. The degree to which transplanted NSCs differentiate into neurons varies by study and NSC type, and includes ranges of 34–46 % for human fetal-derived NSCs [28, 31, 83], 40–66 % for human iPSC-derived NSCs [46, 84], and 30 % for human embryonic-derived NSCs [85]. Evidence of integration by transplanted NSCs includes the expression of synaptic proteins [86], identification of synapse formation with neighboring host neurons by electron microscopy [31, 77], and detection of voltage-gated sodium currents by electrophysiology [46, 77, 85]. While it is unlikely that integration into host circuits by transplanted NSCs can account for the enhanced stroke recovery in these studies, given the delayed timing relative to stroke recovery onset, and low frequency of integration, a remaining question is whether integrated NSCs might instead play a role in the maintenance or progression of long-term functional recovery. And if so, what role would the integrated cells play? The original idea of simple cell replacement and replacement of lost circuits is unlikely, given the low numbers of integrating neurons compared to the vast number of neurons lost after stroke. A more attractive hypothesis is that the integrating neurons influence the excitability of remaining circuits and in doing so affect brain plasticity, which is a major component driving stroke recovery.
Cell integration applies not only to neurons but also to astrocytes and oligodendrocytes that can be derived from NSCs. Astrocytes play a major role in brain plasticity from coordinating brain activity with blood supply and energy, maintaining the blood–brain barrier, to regulating synapse formation and activity [87–90]. While transplanted NSCs have been shown to mature into astrocytes in the stroke-injured brain [29, 77], the relevance of this to stroke recovery requires further investigation. In addition, stroke injury often includes significant damage to white matter, indicating that strategies to replace lost oligodendrocytes and support remyelination could be effective. Remyelination by human NSCs has been demonstrated in the injured spinal cord of rats and mice [23, 91], and some studies have shown oligodendrocyte differentiation by transplanted NSCs in the ischemic brain [21, 33, 77, 78].
Since its initial conception as a cell-replacement strategy, IC transplantation of NSCs is now thought to enhance brain repair and stroke recovery via multiple mechanisms. In the early-phase posttransplantation, it is likely that NSC paracrine signaling plays a critical role in the activation of endogenous repair pathways, such as plasticity, angiogenesis, immunomodulation, and neurogenesis. Subsequent to this, the formation of physical connections between transplanted cells and surrounding host cells may facilitate additional recovery and/or the maintenance of enhanced recovery. Additional preclinical studies that track long-term recovery after stroke will be needed to increase our understanding of this. Nonetheless, an ever-growing list of preclinical studies has shown that IC delivery of NSCs can stimulate brain repair and improve stroke outcome and has allowed advancement into clinical trials.
Advancing to the Clinic: Progress and Remaining Challenges
Clinical Testing of IC-based Cell Therapies for Stroke
To date, very few stroke clinical trials involving IC delivery of NSCs or other human cell types have been approved (Fig. 7.2, Table 7.1), but those that have advanced generally produced encouraging results. IC delivery of NT2-derived neural cells became the first cell therapy to be applied to stroke, with results of the phase I and phase II trials demonstrating the general safety of the IC delivery method and indicating some potential for functional improvement with NT2-derived cells [15, 17, 18].
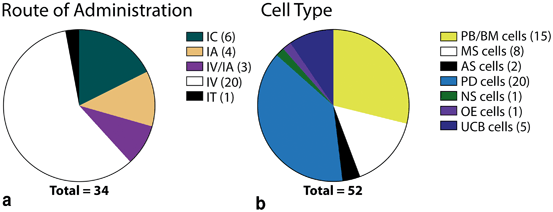
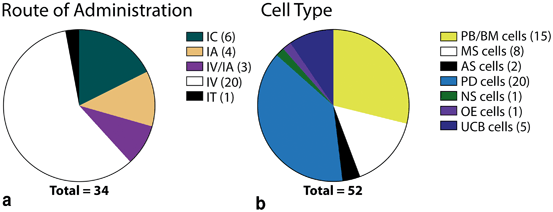
Fig. 7.2
Administration route and cell types used in stroke clinical trials. a Administration routes used in stroke clinical trials. b Cell types used in stroke clinical trials. IC intracerebral, IA intra-arterial, IV intravenous, IT intrathecal, PB/BM cell peripheral blood- or bone marrow-derived cells, MS cells mesenchymal stromal cells, AS cells adipose-derived stromal cells, PD cells placenta-derived cells, NS cells neural stem cells, OE cells olfactory ensheathing cells, UCB cells umbilical cord blood-derived cells
Table 7.1
Registered stroke clinical trials with IC administration of cells
ID | Study phase | Cell type | Administration time post stroke | Cell dose (million) | Recruitment status |
---|---|---|---|---|---|
NCT02117635 | Phase 2 | Human fetal cortical cells (CTX0E03 DP) | > 4 weeks | 20 | Recruiting |
NCT01151124 | Phase 1 | Human fetal cortical cells (CTX0E03 DP) | 6–60 months | 2; 5; 10; 20 | Ongoing, not recruiting |
NCT01714167 | Phase 1 | Autologous bone marrow mesenchymal stem cells | 3–60 months | 2–4 | Recruiting |
NCT00950521 | Phase 2 | Autologous peripheral blood CD34 stem cells | 6–60 months | 2–8 | Completed |
NCT01287936 | Phase 1 Phase 2 | Modified mesenchymal stromal cells (SB623) | 6–60 months | 2.5, 5, 10 | Ongoing, not recruiting |
NCT01327768 | Phase 1 | Olfactory ensheathing cells | 6–60 months | 2–8 | Unknown |
More recently, a conditionally immortalized NSC line developed by Reneuron, CTX0E03, was approved for clinical testing in the UK following promising preclinical safety and efficacy data [92]. The phase I open-label, single-site, dose-ascending trial (PISCES trial) consisted of IC delivery of CTX0E03 into male patients with ischemic stroke 6–60 months after injury at doses of 2, 5, 10, or 20 million cells. Results of this trial recently were presented at the European Stroke Conference, and indicated that as of January 1, 2014, no immunological or cell-related adverse events were observed in any of the 11 patients treated with CTX0E03. Further, CTX0E03-treated patients exhibited functional improvements at 1 month and 1 year postimplantation [93]. Based on these encouraging results, a phase II trial was recently approved to include up to 41 patients treated with CTX0E03 8–12 weeks post stroke. It is expected that this earlier treatment timing better reflects the optimal therapeutic window of CTX0E03, which will be assessed by multiple functional outcome measures up to 6 months posttreatment (www.reneuron.com).
While CTX0E03 is the only IC-administered NSC product currently in clinical testing for stroke, IC delivery of mesenchymal stromal cells (MSCs) is also being clinically tested. SanBio completed the first North American stroke clinical trial to utilize IC delivery of MSCs, specifically testing the human MSC line, SB623. Following confirmation of the safety and efficacy of SB623 in preclinical stroke [94], SanBio initiated a phase II/phase IIa clinical trial testing SB623 delivered IC 6–60 months after ischemic stroke at doses of 2.5, 5, and 10 million cells. Results of this trial recently were presented at the International Stroke Conference and Western Neurosurgical Society, and indicated that as of September 2014, no cell-related adverse events were observed in any of the 18 patients treated with SB623. Three measures of efficacy (NIHSS, ESS, Fugl-Meyer) all demonstrated a statistically significant improvement at 6 months after treatment that continued during follow up to a year. Two patients showed remarkable improvement in their motor (2) and language function (1) within 24 h of surgery, effects which have been sustained during follow-up of 32 and 16 months [95, 96]. Although the mechanisms underlying the functional improvements noted in this trial are not fully defined, these results suggest that the IC cell-transplant surgery can have early beneficial effects on chronic stroke outcome that may persist in the long term.
Remaining Challenges for Clinical Translation
Scaling Up to Meet Clinical Demand
As we continue to explore the potential of NSCs and IC administration as therapeutic strategies for stroke, several challenges remain. First, to meet the demand of the increasingly high number of stroke cases that occur each year (estimated 795,000 new or recurrent stroke cases annually in the USA [97]), improved methods for large-scale production of clinical-grade NSCs must be established. While embryonic-derived NSCs or immortalized cell lines may be the best option for this, given the virtually unlimited scalability of the starting material, they may also pose the greatest safety risk due to the potential for undifferentiated cells to persist in the final product and undergo tumorigenesis or ectopic tissue formation in vivo, particularly when transplanted into the stroke-injured brain [98, 99]. Similarly, though iPSC technologies may ultimately allow reasonable scalability of patient-specific NSCs from a more ethically acceptable source, they will also require strategies to address any undifferentiated cells that remain in the final product [98, 100], as well as the carryover of aberrant genetic or epigenetic changes that can accumulate in aged somatic cells. Alternatively, fetal-derived NSCs pose less tumorigenic risk, but their scalability is more limited, both by the relative availability of appropriate fetal starting material and the extent to which intermediate precursors or the final NSC product can be expanded in vitro. Because extended time in culture can alter a cell’s phenotype and even lead to chromosomal abnormalities, any efforts to scale up NSC production will require careful monitoring of karyotypic stability, as well as the specific molecular signatures that determine an NSC product’s clinical release criteria (California Institute for Regenerative Medicine (CIRM) Disease Team Grant DR1 01480) [40].
Defining Clinical Release Criteria
A second major challenge faced by NSC and non-NSC cell therapies is the need to adequately characterize the final cell product, including quantitative measures of cell identity, purity, and any potential impurities (California Institute for Regenerative Medicine (CIRM) Disease Team Grant DR1 01480) [40]. Together, these measures define key elements of the release criteria that determine the consistency and clinical eligibility of each manufactured lot for a given cell-therapy product. Establishing appropriate quantitative measures of cell identity and purity remains difficult for NSCs, given that surface proteins and intracellular markers typically associated with NSCs either are expressed only transiently during differentiation or are also expressed by other cell types. Use of multiple identity/purity markers can help overcome these limitations, but also can increase the risk of a failed manufacturing run if any of the predetermined release criteria are not met. Furthermore, to ensure safety of an NSC product, its release criteria must also include appropriate impurity markers indicative of unwanted cell types, with acceptable impurity levels ideally based on preclinical studies where the known impurity is spiked into the NSC product at increasing levels and tested for its relative impact on safety. In the case of embryonic- and iPSC-derived NSCs, residual pluripotent cells represent the most concerning type of impurity given their known tumorigenic potential [98, 99], and pluripotency markers such as Oct4, Tra-1-60, and SSEA-4 often are used to detect these cells.
In addition, a detailed understanding of the production/derivation process is necessary to identify any other unwanted cell types that might arise and persist in the final product. An example of this was seen with the first ever clinical trial to test a human embryonic-derived cell therapy, which consisted of embryonic-derived oligodendrocyte progenitor cells (OPCs) developed by Geron Corporation as a direct intraparenchymal spinal treatment for patients with subacute thoracic spinal cord injury. Prior to its initiation, the trial was put on temporary hold after preclinical safety studies indicated the presence of microscopic epithelial cysts in some rats treated with the OPCs [101], thus indicating that this type of NSC product may require detection or removal of epithelial impurities as part of the manufacturing process. Based on a favorable safety profile with the five patients treated to date, it was recently announced that this OPC product, since acquired by Asterias Biotherapeutics, Inc., will advance into a phase I/phase IIa dose escalation study for patients with subacute cervical spinal cord injury (www.asteriasbiotherapeutics.com).
In Vivo Functional Profiling of Transplanted NSCs
Based on guidelines provided by the US Food and Drug Administration (FDA), the development of candidate NSC products must include extensive preclinical testing to sufficiently characterize key functional properties posttransplantation, including their proliferative capacity, differentiation potential, and putative mechanism(s) of action [40, 102]. The proliferative capacity of an NSC product for stroke is an important aspect of its safety profile, as unrestricted growth could lead to transplanted cells spreading beyond the intended graft site or even tumorigenesis. However, most preclinical stroke studies with NSCs indicate very low posttransplant survival and proliferation [21, 31, 51, 52]. Efforts to enhance this, such as hypoxia preconditioning [37, 103] or coadministration of NSCs with some kind of structural support matrix [104, 105], may improve efficacy of NSCs, particularly for large stroke lesions, but whether this will also increase the tumorigenic risk remains to be determined.
As discussed in “Mechanisms of Action for Transplanted NSCs in Stroke,” maximizing the efficacy of NSCs in stroke requires an in-depth understanding of how transplanted NSCs act in the host brain to promote recovery, including their capacity to survive, differentiate, integrate into the host brain, and stimulate endogenous repair through paracrine signaling. For example, the in vivo secretion profile of transplanted NSCs remains largely unknown, but such knowledge could guide development of optimized NSCs that express higher levels of active paracrine factors or enrichment strategies used to purify active subpopulation(s) from an NSC product. It is widely accepted that NSCs are heterogeneous, and this may be advantageous especially if multiple cell types (e.g., neurons, astrocytes, and oligodendrocytes) are required for efficacy. However, if only a certain subpopulation of cells is required (e.g., VEGF-expressing cells or neuronal precursors) then enrichment for this active suppopulation could increase the “effective dose” of the cells.
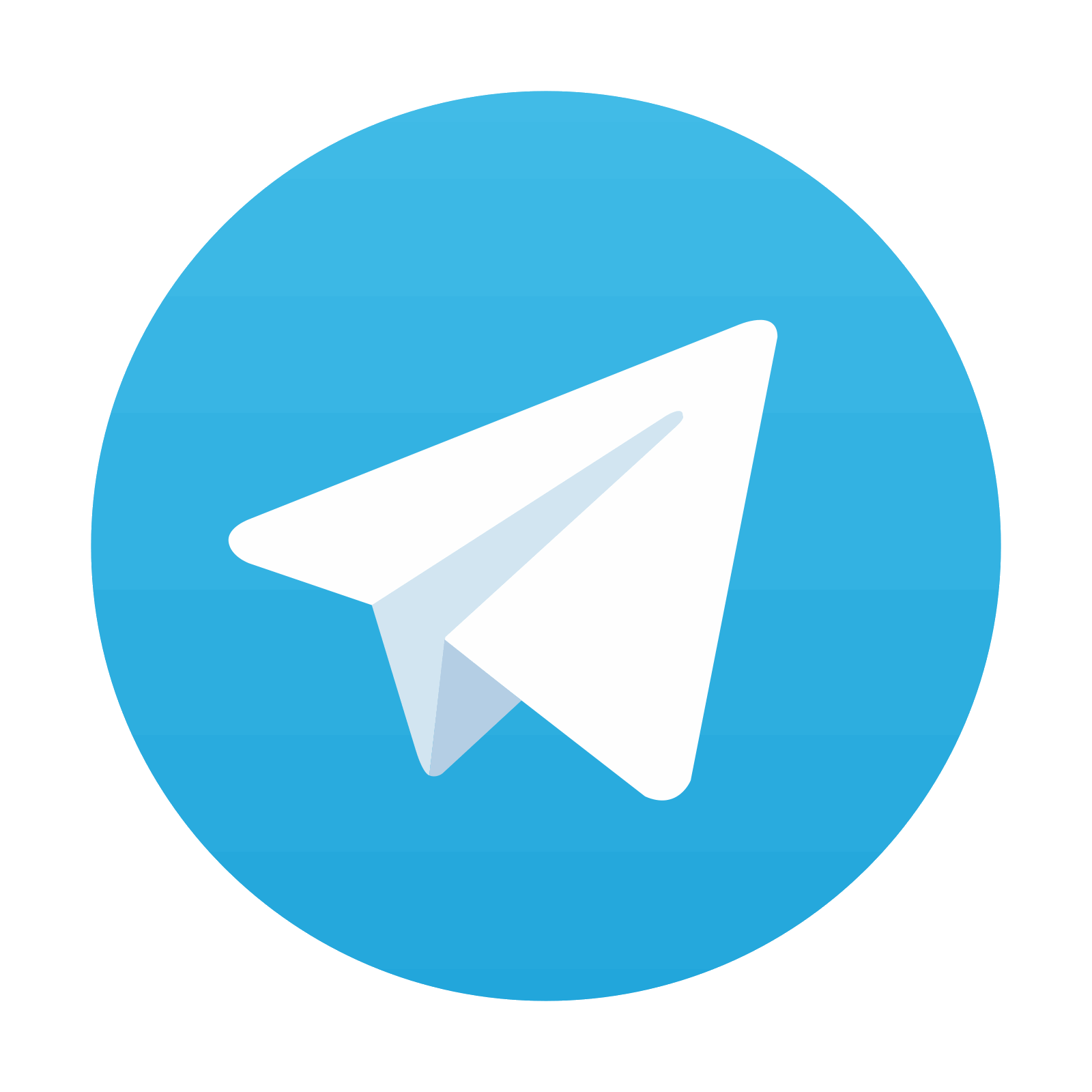
Stay updated, free articles. Join our Telegram channel
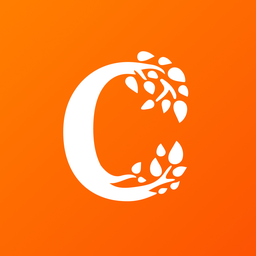
Full access? Get Clinical Tree
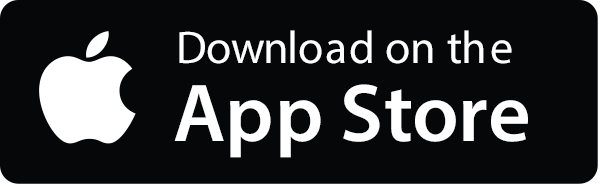
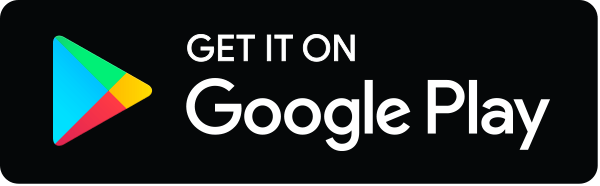