The History and Physical Examination
Thorough and detailed medical history and examination are most important in neuromuscular disorders for diagnosis and proper interpretation of ancillary tests, such as electrodiagnostic studies, imaging or nerve and muscle biopsies, and genetic tests. During the evaluation, the clinician needs to determine if the disease is acquired or inherited and needs to localize the affected regions of the neuromuscular system to find out if the process is focal (i.e., mononeuropathy or radiculopathy) or generalized (i.e., polyneuropathies or myopathies).
Weakness is a common manifestation of neuromuscular disorders, and this could be progressive or of rapid onset, as in Guillain–Barré syndrome (GBS) and rhabdomyolysis, or could fluctuate during the day, as in myasthenia gravis (MG). This could also be intermittent and episodic, as in the periodic paralyses. Weakness is proximal in most myopathies ( Fig. 2-1 ) and some motor neuron diseases and is distal in most neuropathies ( Fig. 2-2 ). There are some dystrophies as well as inclusion body myositis that can present with distal weakness. It could be focal or asymmetric in motor neuron diseases, mononeuropathies, plexopathies, or radiculopathies ( Table 2-1 ).


Clinical Parameter | Motor Neuron Disease | Polyneuropathy | Diseases of Neuromuscular Junction | Myopathy |
---|---|---|---|---|
Pattern of weakness | Variable, symmetric in most, often asymmetric in ALS | Distal > proximal | Proximal > distal, fluctuates, often involves extraocular muscles | Proximal > distal |
Fasciculations | Yes | Sometimes | No | No |
Muscle stretch reflexes | Variable, decreased in most, increased in ALS | Decreased or absent | Normal in postsynaptic disorders (myasthenia gravis), decreased in presynaptic disorders (Eaton–Lambert syndrome and botulism) | Normal initially, may be decreased in later stages (ankle reflexes often preserved until very late) |
Sensory loss | No | Usually present | No | No |
Involvement of the cranial nerves helps in diagnosis. For example, fluctuating ptosis and diplopia could suggest MG, and ptosis, hearing loss, lipomas, and cardiac disease suggest a mitochondrial disorder.
A history of fatigue after physical activity would also suggest a disorder of neuromuscular transmission, whereas exercise intolerance accompanied by pain and contractures suggests a metabolic myopathy as in glycogen storage diseases. Muscle stiffness could suggest myotonia or other disorders of motor unit hyperactivity as in stiff-person syndrome.
Sensory complaints such as pain and numbness are characteristics of diseases affecting the sensory axons in peripheral nerves, roots, or plexus.
A good physical examination will elicit findings that lead to a diagnosis. For example, skeletal abnormalities such as scoliosis or high arches occur in hereditary ataxias and neuropathies, visceromegaly in some polyneuropathies such as the POEMS (polyneuropathy, organomegaly, endocrinopathy, monoclonal gammopathy, and skin changes) syndrome. Mees’ lines in fingernails are seen in arsenic poisoning, while a skin rash is seen in dermatomyositis.
The clinical evaluation should also determine if there is autonomic dysfunction as that occurs in Eaton–Lambert syndrome and some neuropathies.
Gait and posture should be observed in order to detect prominent lordosis seen in stiff-person syndrome and some myopathies, the waddling gait seen in myopathies, or the steppage gait seen in polyneuropathies. The examiner should also note if the patient is able to rise up from the floor or a chair, in those with hip extensor weakness, or is unable to step up to or down from a stool from hip flexor or quadriceps muscle weakness.
The presence of selective, or more prominent, weakness in some muscle groups is helpful. For example, ptosis and extraocular weakness are seen in MG, oculopharyngeal dystrophy, and mitochondrial myopathies. Facial weakness is characteristic of facioscapulohumeral dystrophy but can also be seen in sarcoidosis and familial amyloidosis. Tongue weakness with atrophy and fasciculations occurs in motor neuron disease. Tongue weakness without fasciculations can be seen in MG. A large tongue is seen in older children with Duchenne dystrophy as well as in patients with Pompe disease. Proper examination of palatal movement also helps to determine the risk of aspiration in these patients.
Neck flexor weakness is most common in myopathies, but some patients could also present with a head drop because of neck extensor weakness. This can be seen in, among others, myositis, some dystrophies, amyotrophic lateral sclerosis (ALS), mitochondrial myopathies, and MG. Several myopathies may show characteristics of winging of the scapulae, but when this is unilateral, it could be caused by weakness of the rhomboid, trapezius, or serratus muscles in focal nerve disorders.
Manual muscle testing is always necessary, and the major groups should be quantitated. The grading system used in the cases presented in this book is the standard Medical Research Council scale, which is as follows: 5 = normal strength; 4 = the patient holds the test position against gravity and moderate resistance; 3 = the muscle moves the joint against gravity, but not against resistance to the full extent of mechanical range; 2 = the muscle moves the joint when gravity is eliminated through a partial extent of mechanical range; 1 = there is a flicker of contraction without joint motion; and 0 = no contractions.
The examiner should determine if the weakness is accompanied by focal or generalized atrophy or if there is a lack of significant atrophy as that occurs in disorders of neuromuscular transmission, and frequently in demyelinating neuropathies.
Analysis of muscle strength during repetitive motion helps in diagnosing disorders of neuromuscular transmission, although muscle fatigue can also occur in other diseases.
Muscle stretch reflexes are usually hyperactive in ALS. While distal areflexia is characteristic of axonal neuropathies, generalized areflexia suggests a demyelinating neuropathy. Reflexes are usually normal in myopathies.
Hyporeflexia that improves during repetitive tapping can be observed in Eaton–Lambert syndrome. A focal loss of reflexes, correlating with sensory deficits in territories of nerves, plexuses, or roots, helps in localization.
Reflexes graded in this book are as follows: 0 = absent, 1 = diminished, 2 = normal, 3 = hyperactive normal, and 4 = abnormally increased with clonus. During the examination, the examiner should also determine the presence of Babinski signs in order to diagnose involvement of the corticospinal tracts.
The sensory examination should include the analysis of large myelinated fiber function, such as position and vibratory sense, and the Romberg test and analysis of small fiber function, such as pain and temperature sensations.
Large fiber dysfunction is seen in chronic demyelinating neuropathies, and a preferential impairment of unmyelinated fibers occurs in some neuropathies, like amyloidosis. Touch sensation involves small and large myelinated fibers. The use of the two-point discrimination test and Semmes–Weinstein filaments allows for a better quantitation of touch.
Coordination should be analyzed with the eyes open and closed in order to determine the presence of sensory or cerebellar ataxia.
Important Ancillary Tests
Ancillary tests include measurement of pulmonary function, which can be done in the office with portable respirometers, to determine the forced vital capacity and other respiratory parameters that allow for proper quantitation and follow-up. Other useful tests include electrocardiography and echocardiography in particular disorders such as muscular dystrophies.
Blood tests should include a complete metabolic panel to determine if there is a treatable electrolyte disturbance or renal and liver disease. Elevated enzymes, such as alanine transaminase (ALT), aspartate transaminase (AST), creatine kinase (CK), and aldolase, are seen in myopathies, whereas ALT and AST are also elevated in liver disease, and gamma-glutamyl transpeptidase is elevated only in liver disease. A complete blood count (CBC) should always be done to determine the presence of anemia with macrocytosis which would lead to the diagnosis of pernicious anemia (PA) or folic acid deficiency. Measurement of B 12 and folic acid levels, homocysteine, and methylmalonic acid is used to confirm the diagnosis of PA and folate deficiencies. The CBC is also used in monitoring immunotherapy.
The erythrocyte sedimentation rate can be elevated in acquired autoimmune disorders. Testing for fasting blood sugar and, if necessary, the two-hour postprandial blood sugar test or the glucose tolerance test and measurement of glycosylated hemoglobin help in the diagnosis of diabetic neuropathies.
Other important studies include serum and urine immunoelectrophoresis, using immunofixation to diagnose monoclonal gammopathies, and tests for cryoglobulins in acquired neuropathies. Measurement of arsenic and porphyrins in urine is helpful in patients with neuropathies, whereas myoglobin should be measured in the blood and urine of patients with acute paralysis and elevated serum CK.
An edrophonium test should be performed when disorders of neuromuscular transmission are suspected ( Fig. 2-3 ). If this test is not available, intramuscular prostigmine could be used. The forearm exercise test is useful in diagnosing glycogen storage disease and deaminase deficiency. Specific challenge methods are used, as well, for periodic paralysis.

Antibody Testing
These tests are valuable in the diagnosis of many acquired neuromuscular disorders. For example, stiff-person syndrome is associated with antibodies against glutamic acid decarboxylase, whereas Hμ antibodies, and the recently discovered anti-CV2/CRMP5 antineural antibodies, could be elevated in paraneoplastic neuropathies. Antibodies against various gangliosides in peripheral nerves also help in diagnosis. For example, those against GQ1b ganglioside are associated with Miller Fisher syndrome, while GD1a antibodies can be seen in motor axonal neuropathy. Several are associated with the various forms of GBS and they appear to be pathogenic, including, among others, those against GM1, GM1b, GD1a, glycolipids, and myelin proteins. However, they are not usually measured in the clinical setting.
GD1b antibodies can be elevated in some patients with sensory neuropathies, and those against GM1 ganglioside are frequently high in multifocal motor neuropathy, helping in the diagnosis. A distal demyelinating neuropathy in older persons is frequently associated with antibodies against myelin-associated glycoprotein (MAG). Isaacs syndrome is caused by those that bind the voltage-gated potassium channels in peripheral nerves.
MG is caused by antibodies against the ACh receptors, and patients who are ACh–antibody negative could have them against muscle-specific protein kinase, agrin, or LRP4 antibodies. Eaton–Lambert syndrome is caused by those against the presynaptic voltage-gated Ca channels.
There are several antibodies associated with myositis such as antisynthetase antibodies for which the JO-1 type is particularly important as this can be present in patients with interstitial lung disease. For patients with necrotizing myositis, we should test for signal recognition particle (SRP) antibodies and anti-HMGCR antibodies.
Antinuclear antibodies are seen in lupus erythematosus, and anti- SS-A/Ro or anti- SS-B/La are elevated in Sjögren’s syndrome, a systemic disease which might manifest with a ganglioneuritis or myositis. ANCA titers help in the evaluation of vasculitis. Ganglioneuritis can also occur with neoplasms and anti-Hμ antibodies. Gliadin antibodies help in diagnosing myopathies and neuropathies associated with sprue.
Imaging
Imaging helps to detect structural abnormalities in the spine, plexuses, or even peripheral nerves and to diagnose myeloma associated with neuropathies. Magnetic resonance imaging (MRI) and MR neurography help in the detection of nerve or plexus edema or neoplasm ( Figs. 2-4 and 2-5 ).


MRI of the spine is used to diagnose spinal disease ( Fig. 2-6 ) as shown in several cases in this book and to help rule out a structural disease of the spine that might mimic ALS. MRI can also be used in focal entrapments, for example, to demonstrate structural abnormalities of the carpal tunnel compressing the median nerve. Diagnostic ultrasound is also used with increased frequency in the evaluation of carpal tunnel syndrome (CTS) and other entrapments and muscle atrophy ( Fig. 2-7 ).


Muscle MRI aids in the determination of the presence of inflammation, infarcts, necrosis, or tumors. Inflammation and necrosis are associated with an increased signal in the T2-weighted images ( Fig. 2-8 ), whereas fat replacement is demonstrated in the T1-weighted images ( Fig. 2-9 ). Muscle necrosis also can be shown by radionuclear scanning.


Magnetic spectroscopy is a technique of great value in detecting metabolic myopathies causing muscle fatigue but is now largely used as a research tool.
MRI and ultrasound of muscle help to determine the pattern of muscle involvement in children and to select muscles for biopsies.
Dual-energy x-ray absorptiometry scan is a radiographic method that assesses total-body mineral composition and uses scanners with an x-ray tube with K-edge filtration. Body tissues have varying degrees of absorption ratios, depending on the density, and thus, the technique detects bone mineral content, lean body mass, and fat, and this is used to diagnose osteopenia, measure muscle mass, and determine disease progression in therapeutic trials, having a good correlation with manual muscle testing.
Electrical bioimpedance is a new method that does not provide imaging but analyzes the response of muscles following stimuli of low-intensity electrical currents with a high frequency of 50 kHz. This measures the resistance and reactance of tissue, and the increased capacitance is associated with better muscle mass. The test is done with portable equipment and is simple, safe, rapid, and reproducible. Different techniques are used such as measurement of the total body mass, whereas others study selected muscles. We use regional limb impendence using the Quantum V segmental method which provides appendicular (each exam and leg and torso and total body) composition results and has demonstrated lack of variability when normal subjects are tested twice at different dates. We have used the technique to monitor the progression and response to therapy in neuromuscular diseases.
Clinical Electrophysiologic Studies
Electrophysiologic tests, such as nerve conduction studies, needle electromyography (EMG), and repetitive stimulation tests, are valuable tools in neuromuscular disease. They are particularly helpful in the localization of focal or segmental disorders, such as radiculopathies and nerve entrapments. These should be used as an expansion of a proper neurologic history and examination for interpretation ( Table 2-2 ).
Test | Motor Neuron Disease | Polyneuropathy | Myopathy | Diseases of Neuromuscular Junction |
---|---|---|---|---|
Serum muscle enzymes | Normal or mild elevation | Normal | Increased | Normal |
Nerve conduction studies | Normal or low-amplitude CMAPs, normal SNAPs | Usually slow nerve conduction velocities or low-amplitude CMAPs and SNAPs | Normal | Normal |
Electromyography | Decreased number of motor units, evidence of denervation and reinnervation (large motor units) | Decreased number of motor units, evidence of denervation and reinnervation (large motor units) | Normal number of motor units with short duration and low amplitude, frequently polyphasic | Normal or small motor units, variability of motor unit size and shape |
Repetitive nerve stimulation test | Usually normal, decremental responses can occur | Normal | Normal | Decrement of CMAP at low rate of stimulation, increment at fast rates in presynaptic disorders |
Muscle biopsy | Denervation (atrophic angular and target fibers, fiber-type grouping, group atrophy) | Denervation (atrophic angular and target fibers, fiber-type grouping) | “Myopathic” (necrosis, storage material, inflammation) | Normal or some type II muscle fiber atrophy |
Nerve Conduction Tests
Nerve conduction tests are done using conventional EMG equipment which consists of an oscilloscope with differential amplifiers and signal averaging. The equipment has stimulating units connected to the oscilloscope that triggers its sweeps.
Sensory Conduction Studies.
These tests provide a direct assessment of the function and population of large myelinated sensory axons. The sensory nerve action potential ( SNAP ) represents the summation of the potentials of individual nerve fibers with conduction velocities above 34 m/s that have diameters of about 9 µm. This tests large myelinated sensory axons but not small myelinated or unmyelinated fibers. Near nerve needle recording electrodes allow the study of slow myelinated axons. C fibers can be studied indirectly by measuring the sympathetic skin response that evaluates postganglionic autonomic fibers, and also by using other autonomic tests, and microneurography.
The SNAPs are much smaller than compound muscle action potentials ( CMAPs ) and therefore more sensitive to temporal dispersion with increased distances, particularly using proximal stimulation sites. They are used primarily to test distal nerve segments.
Stimulations should be just above maximal and are done either distally—for example, in the digits and recording at the wrist ( orthodromic conduction )—or proximally—for example, at the wrist and recording at the digits ( antidromic conduction ) ( Fig. 2-10 ). The SNAPs obtained with antidromic stimulation are usually larger. The latency is measured at the initial deflection or at the peak of the negative response. In the past, latency to the peak was used, particularly in the orthodromic method, because it was hard to identify the onset of the SNAP. This can now be done easily with signal averaging, allowing measurement of the conduction velocity of faster axons from the SNAP onset.

Because sensory nerve conduction tests do not include the delay of the neuromuscular junction as in the motor conduction test, the sensory nerve conduction velocity can be calculated directly by dividing the distal latency by the distance or by dividing it by the difference in latencies when more than one site is stimulated. Some report only the latency measurements at set distances, particularly when using orthodromic methods.
The amplitude of the SNAPs is measured from the baseline to the peak of the negative response or from the peak of the negative wave to the peak of the positive wave ( Fig. 2-11 ). Axonal dropout can cause a decreased SNAP amplitude, whereas prolongation of latencies with diminished conduction velocity is caused by demyelination. Demyelinating neuropathies can cause not only slowing but also low-amplitude SNAPs, and even their disappearance, because of increased temporal dispersion.

The duration of the SNAP is measured from the beginning of the negative wave to the end of the negative wave. The area is calculated automatically by newer EMG equipment. The area decreases with dropout of axons from axonal degeneration, and its measurement is more accurate than the amplitude to evaluate for axonal dropout, because the latter might be influenced by temporal dispersion.
Decreased SNAP amplitudes and areas can occur in plexopathies and in focal neuropathies, but usually not in radiculopathies, because the sensory axons originate at the posterior ganglia, which are not affected in the latter disorders, thereby helping in the diagnosis ( Table 2-3 ).
Mononeuropathy | Plexopathy | Radiculopathy | |
---|---|---|---|
Muscle weakness and reflexes | Weakness, decreased reflexes in muscles innervated by single nerves | Weakness or reflexes in muscles innervated by roots from affected plexus but by different nerves | Weakness in muscles innervated by the same root but different nerves |
Sensory deficit | Follows a single nerve territory | Follows a plexus sensory territory | Follows territory of the involved roots |
Limb needle electromyography | Signs of denervation following the territory of one nerve | Signs of denervation in multiple nerves involved in affected plexus area (e.g., lower trunk = hand muscles of ulnar, median nerves) | Signs of denervation in muscles innervated by the same roots or by different nerves |
Paraspinal needle electromyography | No paraspinal muscle denervation | No paraspinal muscle denervation | Paraspinal muscle denervation is common |
Motor nerve | Slow in affected nerve; CMAP amplitude could be decreased when stimulating the affected nerve; conduction block could be seen | Normal (CMAP amplitude could be decreased when stimulating nerves whose axons travel through the affected plexus), slowing across Erb’s point | Normal (CMAP amplitude could be decreased when stimulating nerves whose axons originate in affected roots) |
Sensory-evoked responses | Low amplitude and/or prolonged latency SNAP | Low-amplitude SNAP in nerves whose axons travel through the affected plexus area | Normal SNAPs |
Proximal responses (F-waves, H-reflexes) | Could be slow or absent in affected nerves | Could be slow or absent in nerves from affected plexus area | Could be slow or absent in nerves from affected root |
Mixed Nerve Conduction.
Stimulation over a mixed nerve distally and recording directly in more proximal segments measures mixed nerve conduction, using settings similar to those of sensory conduction tests, and is of value in diagnosing focal entrapments. Palmar stimulation of the ulnar and median nerves and the plantar tibial nerve are mixed nerve conduction techniques. The tests measure the velocity and population of sensory and motor axons directly, but also the 1 alpha afferent axons which are more sensitive to compression.
Quantitative Sensory Testing.
Quantitative sensory tests are psychophysical tests that are used in the determination of sensitivity to pain, temperature, and vibration. These are not electrical tests but help in determining the function of various types of nerve fibers ( Fig. 2-12 ).

Motor Nerve Conduction Tests.
Nerve conduction of peripheral motor nerves is studied by stimulating the nerve distally, and at one or more points proximally, while recording the response in a muscle innervated by that nerve ( Fig. 2-13A ). The stimuli should be supramaximal to allow the stimulation of all motor axons. The motor response is called the CMAP , or M-wave ; this is a biphasic potential with initial negativity.

Stimulations and recordings are done with surface disk electrodes, but needle electrodes can also be used. The cathode of the stimulating electrodes should be over the studied nerve and the anode placed at a distance of 2–3 cm. The cathode of the recording electrode is placed over the belly of the tested muscle and the anode over a tendon, usually about 3–4 cm from the cathode.
Motor nerve conduction tests include the measurement of latency from the stimulus artifact to the onset of the CMAP, as this represents the conduction of the fastest axons. This latency includes not only axonal conduction but also the delays of neuromuscular transmission and depolarization of muscle fibers. The latency from the distal stimulating point is called distal latency or terminal latency and those from proximal points are called proximal latencies . The distal latency can be prolonged in some entrapments, such as that of the median nerve in CTS or the tibial nerve in tarsal tunnel syndrome. It is also prolonged in demyelinating neuropathies.
The amplitude of the negative peak (see Fig. 2-13B ), or of the whole wave (peak to peak), is also measured to detect the conduction block from focal demyelination, causing a drop in amplitude obtained from the proximal stimulation compared to the distal response. Changes in amplitude can also occur in cross-innervations, such as in the Martin–Gruber anastomosis. A decreased amplitude of both the proximal and distal responses can be seen in axonal neuropathies.
Measurement of the duration of the negative wave is useful in the diagnosis of demyelination which causes a prolonged CMAP. Such a potential might appear dispersed because of different rates of slowing of the axons. The area of the CMAP is measured automatically with newer equipment; this represents the combination of the amplitude and duration and is more accurate in the assessment of conduction block.
Motor nerve conduction velocity is calculated by subtracting the latency from two stimulating points (see Fig. 2-13C ). For example, if the CMAP is obtained when the median nerve is stimulated at the wrist ( distal stimulation ) and recorded at the thenar eminence, and is also stimulated at the elbow ( proximal stimulation ) using the same recording site, then the distance between the two stimulating points (elbow to wrist) is measured, and the velocity is calculated by dividing the distance by the latency difference. Stimulation of more proximal segments helps to determine focal slowing, such as in ulnar nerve entrapment at the elbow.
Causes of error in motor and sensory nerve conduction tests include measurements at low body temperature which slows conduction velocity. Inappropriate stimulation sites and improper positioning of electrodes can also cause erroneous results. Age and body size play a role in conduction velocity because very old, very young, and very tall individuals have slower conduction velocities.
Demyelination causes slowing of conduction velocity, usually below 80% of normal or lower. Nonuniform nerve conduction slowing is usually seen in acquired neuropathies, whereas uniform slowing occurs in most hereditary demyelinating neuropathies. Acquired demyelinating neuropathies can also cause conduction block and temporal dispersion, with prolongation of the CMAP from the variability of conduction of the various fibers. Demyelination also causes prolongation of the distal latencies (see Fig. 2-13D ), F-wave, and H-reflexes, which could be absent. Several criteria of slowing and conduction block are used in research to diagnose demyelination, as discussed in individual cases of this book.
The terminal latency index ( TLI ) is the ratio of the calculated over the measured latency; it is used to demonstrate prominent demyelination in distal segments, relative to proximal segments, in patients with peripheral neuropathies. A low index indicates disproportionally distal demyelination. This is particularly useful in distinguishing CTS in patients with neuropathies.
The TLI is calculated by determining first the calculated distal latency , which is the latency that corresponds to the recorded proximal conduction velocity; this is obtained by dividing the value of the measured distal distance by the proximal conduction velocity . For example, if the distal distance is 8 cm and the proximal conduction velocity is 50 m/s, the calculated latency will be 1.6 ms (8 cm divided by 50 ms). The TLI is then obtained by dividing the value of the calculated latency by the measured latency . In this example, if the measured latency was 4 ms, the latency index would be 0.4 (1.6/4).
In axonal neuropathies, the CMAP could be of low amplitude, with normal or only mildly decreased velocities, and have normal or mildly decreased latencies. Focal nerve lesions, such as neurapraxia (first-degree lesions of Sunderland classification), are caused by edema and focal demyelination, showing conduction block from stimulation above to the lesion. Its recovery time can vary from hours to a few months.
Axonotmesis describes a lesion of the axon causing its degeneration but maintaining the integrity of the connective tissue. This can be subdivided into three types: (1) when the axon is discontinued but the endoneurium is intact (second degree of Sunderland); (2) when the axon and endoneurium are discontinued, but the perineurium and epineurium are preserved (third degree of Sunderland); and (3) when the epineurium remains intact (fourth degree of Sunderland). A lesion affecting the axon and its connective tissue, when the nerve is completely severed, is called neurotmesis (fifth degree of Sunderland).
In axonotmesis and neurotmesis the CMAP obtained from stimulation below the lesion remains normal until enough time has elapsed to produce a complete axonal degeneration, but no CMAP is obtained upon proximal stimulation. These findings are accompanied by signs of denervation on EMG, and no elicitable motor units in muscles that are innervated by the affected nerve.
The H-Reflex.
This is a late reflex, named after Johann Hoffmann who first described the wave. The H-reflex is obtained by stimulating mixed nerves inframaximally in order to stimulate only sensory axons that have a lower threshold of activation. This stimulus produces a reflex, as the impulse travels orthodromically through the sensory axons, stimulating the posterior root ganglia and then the anterior horns, which depolarizes and produces an electrical impulse. This wave travels orthodromically through its motor axon, producing the late response in muscle ( Fig. 2-14 ). The H-reflex is a monosynaptic reflex, which disappears with the sectioning of the posterior roots in animals.

The stimulus duration used to test the H-reflex should be 0.5–1 ms, as this preferentially stimulates the 1 alpha fibers. During H-reflex tests, either no direct CMAP (or M-wave) is seen or it is very small (as some motor axons might also be stimulated), but the reflex should always be of higher amplitude than the M-wave and have a persistent latency and voltage during sequential stimulation in the same site and in using the same intensity. The H-reflex is usually studied by stimulating the tibial nerve at the knee while recording at the soleus muscle. This has a latency of about 30–35 ms. The reflex can be obtained by stimulating different nerves in young children. In adults this can be studied in the femoral and radial nerves. The tibial H-reflex is useful in diagnosing S1 radiculopathy, in which the reflex could be slow or absent and could also be slow or absent in sciatic, proximal tibial neuropathies, and lumbosacral plexopathies. This is usually, but not always, absent when the ankle reflex disappears. Absent H-reflexes are the earliest findings in GBS.
The F-Response.
This is a late response whose name comes from foot where it was initially recorded. The wave is obtained by supramaximal stimulation of a motor nerve. This stimulus travels orthodromically producing an early CMAP and also travels antidromically toward the spinal cord stimulating motor neurons. Upon depolarization, these neurons send an electrical impulse that travels orthodromically back to the muscle, producing a late motor action potential ( Figs. 2-15 and 2-16 ). Each F-wave represents a stimulated individual motor unit. They vary in shape and latency, but not all stimulations produce an F-wave ( Fig. 2-17 ).



Supramaximal stimuli also depolarize the sensory axons, but this orthodromic response collides with the motor response.
The F-wave normally has a latency of about 28–30 ms recorded in the hand; in the foot, the latency is 40–55 ms. The individual F-waves are obtained with each stimulation, vary in latency, and are much smaller than the M-wave. Their latency represents the motor conductions of individual motor unit axons.
Conventionally, the shortest latency obtained from several stimulations is measured. Some electromyographers use F-latencies to calculate their conduction velocities. The latency could be prolonged in peripheral neuropathies and focally in radiculopathies and plexopathies. They tend to disappear or be prolonged in demyelinating neuropathies. Normally, about 50% of the waves are obtained from various direct motor stimuli, and the number of F-waves (F-wave persistency) is usually diminished in demyelinating neuropathies ( Figs. 2-17 and 2-18 ).

Increased variations of F-wave latencies are seen in demyelinating neuropathies ( chronodispersion ) from variations of conductions of individual axons. The variation in conduction velocities is called tachydispersion .
The Axonal Wave.
The axonal (A) wave is a response observed sometimes during F-wave studies, and its latency is usually shorter than that of the F-waves ( Fig. 2-19 ), although occasionally they appear late. A-waves persist during most or all sequential stimulations and are of identical shape and latency. The waves are believed to be caused by ephaptic or ectopic discharges of demyelinating axons but may also be caused by axonal sprouting in which the antidromic stimuli activate the regenerating axon.

Other Nerve Conduction Tests Used in Neuromuscular Disorders.
Somatosensory-evoked responses and motor responses obtained during magnetic stimulation allow the study of sensory and motor pathways, not only of the brain and spinal cord but also of peripheral nerves; these are used in the diagnosis of proximal nerve, plexus, or root damage. Proximal nerve segments can also be studied by direct root stimulation. Magnetic stimulation of the brain is used to study motor pathways.
Routine Needle Electromyography
Routine EMG is performed with needle electrodes to record the electrical characteristics of the motor unit action potentials (MUAPs) as seen in the oscilloscope and to observe for abnormal spontaneous potentials. Analysis of the sound of these potentials is also of value; this is done with the use of a loudspeaker attached to the oscilloscope.
EMG helps in the diagnosis of generalized disorders and to localize segmental diseases. For example, mapping the myotomal distribution of denervation potentials allows the examiner to localize the affected root and assist in the differentiation of radiculopathy from plexopathies or mononeuropathies.
Recordings are done using either bipolar or monopolar needle electrodes. Bipolar needle electrodes have the anode and the cathode in their tip concentric electrodes, the cathode in the center, and the reference in the shaft. Monopolar needle electrodes, which are Teflon coated, have the cathode in the uncovered tip; a distant surface electrode is used for reference. The characteristics of the recorded potentials vary with the needle. For example, recording with monopolar needles produces more artifacts, a less stable baseline, and higher amplitude motor units with an increased number of polyphasic MUAPs, compared with recordings obtained with concentric electrodes.
During routine EMG, the following are analyzed.
Insertional Activity.
The insertion of the needle electrode in the muscle causes a mechanical stimulation that produces discharges that are called insertional activity ( IA ). This burst of electrical potentials indicates that the needle is indeed in the muscle. The IA has increased the duration in denervated and necrotic muscles and myotonias. It is decreased if the muscle is replaced by connective tissue or fat. The IA is also decreased during attacks of periodic paralysis or contractures in McArdle’s disease, from decreased excitability of the muscle membrane.
After analysis of the IA, the muscle is examined at rest, and, normally, there should be no electrical activity, unless the needle is placed next to the endplate, where there are variations of the baseline or endplate noise . Endplate noise is caused by extracellular recordings of miniature endplate potentials (EPPs). Endplate spike potentials can also be observed; these are of negative onset biphasic waves which are repetitive and of continuous discharges of individual muscle fibers firing irregularly (5–50 Hz with amplitudes of 100–200 μV) ( Fig. 2-20 ).

Abnormal Spontaneous Potentials.
Fasciculations are spontaneous depolarizations of individual motor units ( Fig. 2-21 ). They usually produce visible movements of the electrode. Fasciculations can occur in healthy people, but when accompanied by muscle atrophy and weakness, they usually indicate a disorder of the motor neuron. Fasciculations, however, can also occur in radiculopathies, in some neuropathies, in some metabolic disorders, and in anticholinesterase overmedication.

Differentiation of normal fasciculations from those of neuromuscular disorders is difficult, but in neurogenic disorders, which cause large and polyphasic voluntarily elicited MUAPs, the fasciculations might also show these characteristics. The firing rate is faster in normals than in those with neurogenic disorders, but this is difficult to assess and not reliable in diagnosis.
Myokymic discharges are the spontaneous firing of motor units which usually contain 2–10 spikes discharging at 30–40 Hz in each burst. They occur at regular intervals and have the characteristic sound of “marching soldiers” ( Fig. 2-22 ). Myokymic discharges can be seen in radiculopathies and in plexopathies, particularly those caused by radiation. These are also seen in GBS and in the facial muscles of multiple sclerosis and brainstem gliomas.

Fibrillations are spontaneous biphasic potentials of about 1–5 ms duration and of 20–500 μV amplitude ( Figs. 2-23 and 2-25 ). They usually have an initial negative phase, except when the needle is close to the endplate, in which case they can have a positive onset and may be difficult to differentiate from endplate spikes. Fibrillations are spontaneous depolarizations of individual muscle fiber; these depolarizations are caused by increased sensitivity to acetylcholine (ACh) from diffuse extrajunctional ACh receptors in denervated or necrotic muscle fibers.


Fibrillations usually appear 2 weeks after a nerve injury, but their onset depends on the distance from the site of the injury to the studied muscles. Thus, they appear earlier in more proximal muscles, where they also disappear earlier during recovery. They are smaller in more chronic disorders.
These potentials are characteristically seen in neurogenic disorders but also occur in necrotizing myopathies, particularly polymyositis ( Fig. 2-25 ) and muscular dystrophies. The presence of fibrillations in different muscle groups helps the electromyographer to localize segmental diseases (see Table 2-3 ).
Positive sharp waves are monophasic or biphasic potentials that represent fibrillations that have lost their negative spike, caused by trauma from the needle electrode. They have the same significance as fibrillations and are caused by denervation but are also seen in other disorders. They usually have a sawtooth appearance with initial positivity that could be followed by a slower negative wave (see Figs. 2-24 and 2-25 ). These can be seen during needle insertions or movements but also fire spontaneously.

Neuromyotonia are fast-firing potentials that originate in the peripheral nerve with a frequency of 100–300 Hz. They have a typical high-pitched “pinging” sound and decline in amplitude very quickly. These can be caused by nerve injuries and are recorded intraoperatively when nerves are stretched. Neuromyotonic discharges can be seen in spinal muscular atrophies (SMAs), in some neuropathies, and in Isaacs syndrome accompanied by other spontaneous discharges.
Complex repetitive discharges ( CRDs ) are discharges that have an abrupt onset and disappearance and do not fluctuate in amplitude, remaining unchanged ( Fig. 2-26 ). These complex and polyphasic waves are formed by several individual fiber potentials. The group fires at 50–100 ms and has amplitudes of 50 μV–1 mV. CRDs are caused by ephaptic transmission of individual muscle fibers that fire nearly synchronously. They are seen in neurogenic diseases but also in myopathies, such as polymyositis, acid maltase deficiency, some muscular dystrophies, and Schwartz–Jampel syndrome.

Myotonic discharges are potentials of amplitude from 10 μV to 1 mV and vary in duration and amplitude, waxing, and waning; they do not disappear abruptly ( Fig. 2-27 ). They are characteristically seen in myotonic disorders but also occur in other channelopathies, such as hyperkalemic periodic paralysis, polymyositis, and acid maltase deficiency. Myotonia is caused by abnormalities of the muscle chloride or the sodium channels, which increase membrane excitability.

Cramps are sustained involuntary contractions of nerve origin that are not electrically silent, unlike the muscle contractures seen in metabolic myopathies. The cramp potentials consist of repetitive discharges of normal-appearing motor units, which fire very rapidly at 200–300 Hz.
Motor Unit Action Potentials.
MUAPs are produced by the summation of electrical potentials of individual muscle fibers innervated by the same motor neuron and are activated by voluntary muscle contractions. The recorded MUAP represents potentials of about 8–10 fibers that are close to the needle electrode ( Fig. 2-28 ).
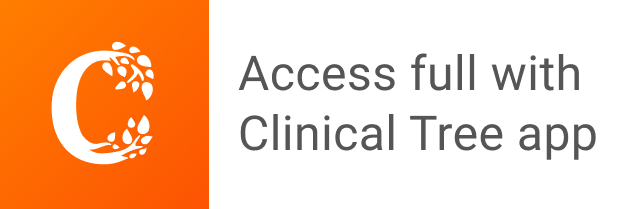